The Oral Environment
The tooth contains three specialized calcified tissues: enamel, dentin, and cementum (Figure 2-1). Enamel is unique in that it is the most highly calcified tissue in the body and contains the least organic content of any of these tissues. Enamel provides the hard outer covering of the crown that allows efficient mastication. Dentin and cementum, like bone, are vital, hydrated, biological composite structures formed mainly from a collagen type I matrix reinforced with the calcium phosphate mineral called apatite. Dentin forms the bulk of the tooth and is joined to the enamel at the dentin-enamel junction (DEJ). The dentin of the tooth root is covered by cementum that provides connection of the tooth to the alveolar bone via the periodontal ligament. Although the structure of these tissues is often described in dental texts, the properties are often discussed only superficially. However, these properties are important in regard to the interrelationships of the factors that contribute to the performance necessary for the optimum function of these tissues.
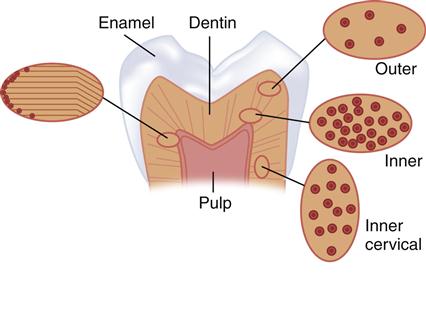
On the right side are illustrations of dentin tubules as viewed from the top, which shows the variation in the tubule number with location. At the left is an illustration of the change in direction of the primary dentin tubules as secondary dentin is formed. (From Marshall SJ, et al: Acta. Mater. 46, 2529-2539, 1998.)
In restorative dentistry we are interested in providing preventive treatments that will maintain tissue integrity and replace damaged tissues with materials that ideally will mimic the natural appearance and performance of those tissues when necessary. Thus knowledge of the structure and properties of these tissues is desirable both as a yardstick to measure the properties and performance of restorative materials and as a guide to the development of materials that will mimic their structure and function. In addition, many applications, such as dental bonding, require us to attach synthetic materials to the calcified tissues, and these procedures rely on detailed knowledge of the structure and properties of the adhesive tissue substrates.
Enamel
Figure 2-1 shows a schematic diagram of a posterior tooth sectioned to reveal the enamel and dentin components. Enamel forms the hard outer shell of the crown and as the most highly calcified tissue is well suited to resisting wear due to mastication.
Enamel is formed by ameloblasts starting at the dentin-enamel junction (DEJ) and proceeding outward to the tooth surface. The ameloblasts exchange signals with odontoblasts located on the other side of the DEJ at the start of the enamel and dentin formation, and the odontoblasts move inward from the DEJ as the ameloblasts forming enamel move outward to form the enamel of the crown. Most of the enamel organic matrix composed of amelogenins and enamelins is resorbed during tooth maturation to leave a calcified tissue that is largely composed of mineral and a sparse organic matrix. The structural arrangement of enamel forms keyhole-shaped structures known as enamel prisms or rods that are about 5 μm across as seen in Figure 2-2.
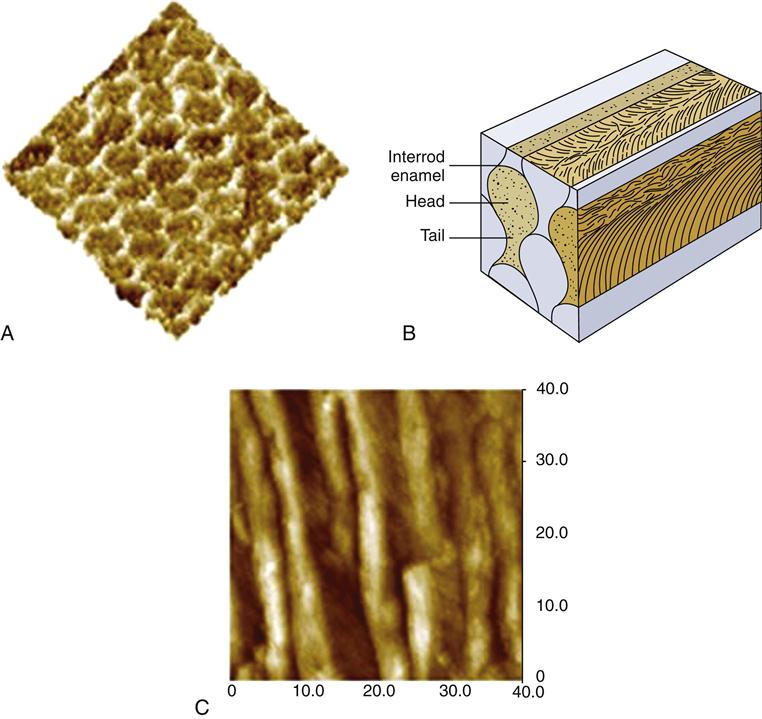
The overall composition is about 96% mineral by weight, with 1% lipid and protein and the remainder being water. The organic portion and water probably play important roles in tooth function and pathology, and it is often more useful to describe the composition on a volume basis. On that basis we see the organic components make up about 3% and water 12% of the structure. The mineral is formed and grows into very long crystals of hexagonal shape about 40 nm across; these have not been synthetically duplicated. There is some evidence that the crystals may span the whole enamel thickness, but this is difficult to prove because most preparation procedures lead to fracture of the individual crystallites. It appears that they are at least thousands of nanometers long. If this is true, then enamel crystals provide an extraordinary “aspect” ratio (length to width ratio) for a nanoscale material, and they are very different from the much smaller dentin crystals. The crystals are packed into enamel prisms or rods that are about 5 μm across as shown in Figure 2-2. These prisms are revealed easily by acid etching and extend in a closely packed array from the DEJ to the enamel surface and lie roughly perpendicular to the DEJ, except in cuspal areas where the rods twist and cross, known as decussation, which may increase fracture resistance. About 100 crystals of the mineral are needed to span the diameter of a prism, and the long axes of the crystals tend to align themselves along the prism axes, as seen in Figure 2-2.
The crystals near the periphery of each prism deviate somewhat from the long axis toward the interface between prisms. The deviation in the tail of the prism is even greater. The individual crystals within a prism are also coated with a thin layer of lipid and/or protein that plays important roles in mineralization, although much still remains to be learned about the details. Recent work suggests that this protein coat may lead to increased toughness of the enamel. The interfaces between prisms, or inter-rod enamel, contain the main organic components of the structure and act as passageways for water and ionic movement. These areas are also known as prism sheaths. These regions are of vital importance in etching processes associated with bonding and other demineralization processes, such as caries.
Etching of enamel with acids such as phosphoric acid, commonly used in enamel bonding, eliminates smear layers associated with cavity preparation, dissolves persisting layers of prismless enamel in deciduous teeth, and differentially dissolves enamel crystals in each prism. The pattern of etched enamel is categorized as type 1 (preferential prism core etching, Figure 2-2, A); type 2 (preferential prism periphery etching, Figure 2-3, C), and type 3 (mixed or uniform). Sometimes these patterns appear side by side on the same tooth surface (Figure 2-3, E). No differences in micromechanical bond strength of the different etching patterns have been established. In a standard cavity preparation for a composite, the orientation of the enamel surfaces being etched could be perpendicular to enamel prisms (perimeter of the cavity outline), oblique cross section of the prisms (beveled occlusal or proximal margins), and axial walls of the prisms (cavity preparation walls). During the early stages of etching, when only a small amount of enamel crystal dissolution occurs, it may be difficult or impossible to detect the extent of the process. However, as the etching pattern begins to develop, the surface etched with phosphoric acid develops a frosty appearance (Figure 2-3, B), which has been used as the traditional clinical indicator for sufficient etching. This roughened surface provides the substrate for infiltration of bonding agents that can be polymerized after penetration of the etched enamel structure so that they form micromechanical bonds to the enamel when polymerized. With self-etching bonding agents, this frosty appearance cannot be detected.
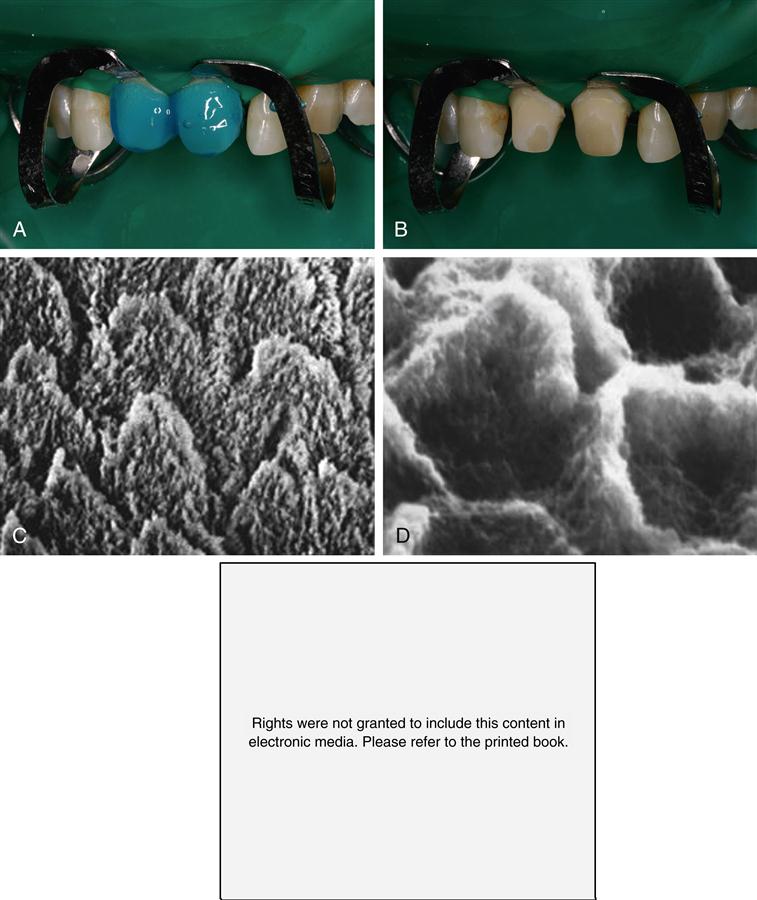
Etching enamel. A, Gel etchant dispensed on the enamel portion of the preparation. B, Frosty appearance after etching, rinsing and drying. C, Magnified view of etch pattern with preferential prism periphery etch (type 1). D, Bonding agent revealed after dissolving enamel. E, Mixed etch patterns showing type 1 (light prisms with dark periphery) and type 2 (dark cores with light periphery) etching on same surface after Marshall et al, 1975 JDR. Marshall GW, Olson LM, Lee CV: SEM Investigation of the variability of enamel surfaces after simulated clinical acid etching for pit and fissure sealants, J Dent Res 54:1222–1231, 1975. Part C from Marshall, Olson and Lee, JDR 1975 (same as above) and Part E from Marshall, Marshall and Bayne, 1988: Marshall GW, Marshall SJ, Bayne SC: Restorative dental materials: scanning electron microscopy and x-ray microanalysis, Scanning Microsc 2:2007–2028, 1988.
Rights were not granted to include this figure in electronic media. Please refer to the printed book.
There are two other important structural variations of enamel. Near the DEJ the enamel prism structure is not as well developed in the very first enamel formed, so that the enamel very close to the DEJ may appear aprismatic or without the prism like structure. Similarly, on the outer surface of the enamel, at completion of the enamel surface, the ameloblasts degenerate and leave a featureless layer, called prismless enamel, on the outer surface of the crown. This layer is more often observed in deciduous teeth and is often worn off in permanent teeth. However, if present, this causes some difficulty in getting an effective etching pattern and may require roughening of the surface or additional etching treatments. The outer surface of the enamel is of great clinical significance because it is the surface subjected to daily wear and undergoes repeated cycles of demineralization and remineralization. As a result of these cycles, the composition of the enamel crystals may change, for example, as a result of exposure to fluoride. Thus the properties of the enamel might be expected to vary from the external to the internal surface. Such variations, including a thin surface veneer of fluoride-rich apatite crystals, create differences in the enamel properties within the enamel. Enamel is usually harder at the occlusal and cuspal areas and less hard nearer the DEJ. Figure 2-4 shows an example of the difference in hardness.
The Mineral
The mineral of all calcified tissues is a highly defective relative of the mineral hydroxyapatite, or HA. The biological apatites of calcified tissues are different than the ideal HA structure in that the defects and chemical substitutions generally make it weaker and more soluble in acids. Hydroxyapatite has the simple formula Ca10(PO4)6(OH)2, with an ideal molar ratio of calcium to phosphorus (Ca/P) of 1.67 and a hexagonal crystal structure. The apatite of enamel and dentin has a much more variable composition that depends on its formative history and other chemical exposures during maturity. Thus the mineral in enamel and dentin is a calcium-deficient, carbonate-rich, and highly substituted form related to HA. Metal ions such as magnesium (Mg) and sodium (Na) may substitute for calcium, whereas carbonate substitutes for the phosphate and hydroxyl groups. These substitutions distort the structure and make it more soluble. Perhaps the most beneficial substitution is the fluorine (F) ion, which substitutes for the hydroxyl group (OH) in the formula and makes the structure stronger and less soluble. Complete substitution of F for (OH) in hydroxyapatite yields fluoroapatite mineral, Ca10(PO4)6(F)2, that is much less soluble than HA or the defective apatite of calcified tissues. It is worth noting that HA has attracted considerable attention as an implantable calcified tissue replacement. It has the advantage of being a purified and stronger form of the natural mineral and releases no harmful agents during biological degradation. Its major shortcoming is that it is extremely brittle and sensitive to porosity or defects and therefore fractures easily in load-bearing applications.
The approximate carbonate contents of the enamel and dentin apatites are significantly different, about 3% and 5% carbonate, respectively. All other factors being equal, this would make the dentin apatite more soluble in acids than enamel apatite. Things are not equal, however, and the dentin apatite crystals are much smaller than the enamel crystals. This means that the dentin crystals present a higher surface area to attacking acids and contain many more defects per unit volume and thus exhibit considerably higher solubility. Finally, as discussed further below, the dentin mineral occupies only about 50% of the dentin structure, so there is not as much apatite in the dentin as there is in enamel. All of these factors multiply the susceptibility of dentin to acid attack and provide insight into the rapid spread of caries when it penetrates the DEJ.
Dentin
Dentin is a complex hydrated biological composite structure that forms the bulk of the tooth. Furthermore, dentin is modified by physiological, aging, and disease processes that result in different forms of dentin. These altered forms of dentin may be the precise forms that are most important in restorative dentistry. Some of the recognized variations include primary, secondary, reparative or tertiary, sclerotic, transparent, carious, demineralized, remineralized, and hypermineralized. These terms reflect alterations in the fundamental components of the structure as defined by changes in their arrangement, interrelationships, or chemistry. A number of these may have important implications for our ability to develop long-lasting adhesion or bonds to dentin.
Primary dentin is formed during tooth development. Its volume and conformation, reflecting tooth form, vary with the size and shape of the tooth. Dentin is composed of about 50 volume percent (vol%) carbonate-rich, calcium-deficient apatite; 30 vol% organic matter, which is largely type I collagen; and about 20 vol% fluid, which is similar to plasma. Other noncollagenous proteins are thought to be involved in dentin mineralization and other functions such as controlling crystallite size and orientation; however, these functions are not discussed further in this text. The major components are distributed into distinctive morphological features to form a vital and complex hydrated composite in which the morphology varies with location and undergoes alterations with age or disease.
The tubules, one distinct and important feature of dentin, represent the tracks taken by the odontoblastic cells from the DEJ or cementum at the root to the pulp chamber and appear as tunnels piercing the dentin structure (Figure 2-5). The tubules converge on the pulp chamber, and therefore tubule density and orientation vary from location to location (see Figure 2-1). Tubule number density is lowest at the DEJ and highest at the predentin surface at the junction to the pulp chamber, where the odontoblastic cell bodies lie in nearly a close-packed array. Lower tubule densities are found in the root. The contents of the tubules include odontoblast processes, for all or part of their course, and fluid. The extent of the odontoblast process is still uncertain, but evidence is mounting that it extends to the DEJ. For most of its course, the tubule lumen is lined by a highly mineralized cuff of peritubular dentin roughly 0.5 to 1 μm thick (Figure 2-6). Because the peritubular dentin forms after the tubule lumen has been formed, some argue that it may be more properly termed intratubular dentin and contains mostly apatite crystals with little organic matrix. A number of studies have concluded that the peritubular dentin does not contain collagen, and therefore might be considered a separate calcified tissue. The tubules are separated by intertubular dentin composed of a matrix of type I collagen reinforced by apatite (see Figures 2-5 and 2-6). This arrangement means that the amount of intertubular dentin varies with location. The apatite crystals are much smaller (approximately 5 × 30 × 100 nm) than the apatite found in enamel and contain 4% to 5% carbonate. The small crystallite size, defect structure, and higher carbonate content lead to the greater dissolution susceptibility described above.
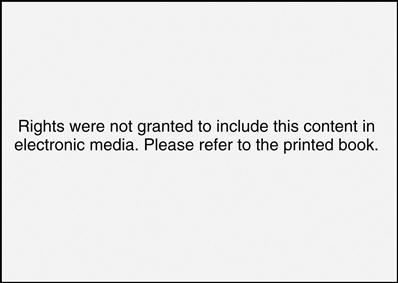
At the top is a view of the tubules, each of which is surrounded by peritubular dentin. Tubules lie between the dentin-enamel junction (DEJ) and converge on the pulp chamber. The perpendicular surface at the bottom shows a fracture surface revealing some of the tubules as they form tunnel-like pathways toward the pulp. The tubule lumen normally contains fluid and processes of the odontoblastic cells.
Rights were not granted to include this figure in electronic media. Please refer to the printed book. (From Marshall GW: Quintessence Int. 24, 606-617, 1993.)
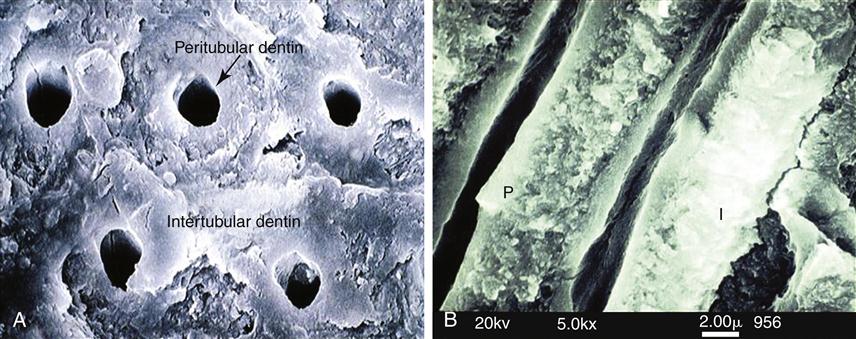
Peritubular (P) (also called intratubular) dentin forms a cuff or lining around each tubule. The tubules are separated from one another by intertubular dentin (I). (Courtesy of G. W. Marshall.)
Estimates of the size of tubules, the thickness of the peritubular region, and the amount of intertubular dentin have been made in a number of studies. Calculations for occlusal dentin as a function of position from these data show the percent tubule area and diameter vary from about 22% and 2.5 μm near the pulp to 1% and 0.8 μm at the DEJ. Intertubular matrix area varies from 12% at the predentin to 96% near the DEJ, whereas peritubular dentin ranges from over 60% down to 3% at the DEJ. Tubule densities are compared in Table 2-1 based on work by various investigators. It is clear that the structural components will vary considerably over their course, and necessarily result in location-dependent variations in morphology, distribution of the structural elements, and important properties such as permeability, moisture content, and available surface area for bonding and may also affect bond strength, hardness, and other properties.
TABLE 2.1
Comparison of Mean Numerical Density of Tubules in Occlusal Dentin∗
Outer Dentin | Middle Dentin | Inner Dentin |
15,000/mm2 | 35,000/mm2 | 65,000/mm2 |
20,000/mm2 | 35,000/mm2 | 43,000/mm2 |
24,500/mm2 | 40,400/mm2 | 51,100/mm2 |
18,000/mm2 | 39,000/mm2 | 52,000/mm2 |
∗From data reported in the literature (Marshall GW: Quintessence Int. 24, 606-617, 1993.)
Because the odontoblasts come to rest just inside the dentin and line the walls of the pulp chamber after tooth formation, the dentin-pulp complex can be considered a vital tissue. This is different than mature enamel. Over time secondary dentin forms and the pulp chamber gradually becomes smaller. The border between primary and secondary dentin is usually marked by a change in orientation of the dentin tubules. Furthermore, the odontoblasts react to form tertiary dentin in response to insults such as caries or tooth preparation, and this form of dentin is often less well organized than the primary or secondary dentin.
Early enamel carious lesions may be reversed by remineralization treatments. However, effective remineralization treatments are not yet available for dentin and therefore the current standard of care dictates surgical intervention to remove highly damaged tissue and then restoration as needed. Thus it is important to understand altered forms of dentin and the effects of such clinical interventions.
When dentin is cut or abraded by dental instruments, a smear layer develops and covers the surface and obscures the underlying structure (Figure 2-7). The bur cutting marks are shown in Figure 2-7, A, and at higher magnification in Figure 2-7, B. Figure 2-7, C, shows the smear layer thickness from the side and the development of smear plugs as the cut dentin debris is pushed into the dentin tubule lumen. The advantages and disadvantages of the smear layer have been extensively discussed for several decades. It reduces permeability and therefore aids in maintaining a drier field and reduces infiltration of noxious agents into the tubules and perhaps the pulp. However, it is now generally accepted that it is a hindrance to dentin bonding procedures and, therefore, is normally removed or modified by some form of acid conditioning.
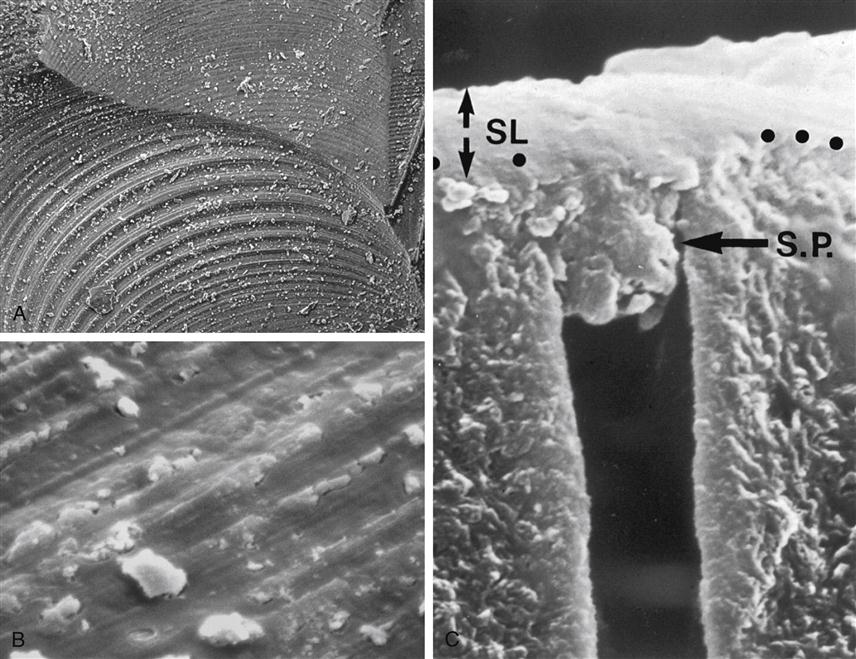
A, Bur marks on dentin preparation B, Higher magnification showing smear layer surface and cutting debris. C, Section showing smear layer (SL) and smear plugs (S.P.). (A and B from Marshall GW, et al: Scanning Microsc. 2, 2007-2028, 1988; C from Pashley DH, et al: Arch. Oral Biol. 33, 265-270, 1988.)
Acid etching or conditioning allows for removal of the smear layer and alteration of the superficial dentin, opening channels for infiltration by bonding agents. Figure 2-8 shows what happens in such an etching treatment. The tubule lumens widen as the peritubular dentin is preferentially removed because it is mostly mineral with sparse protein. The widened lumens form a funnel shape that is not very retentive.
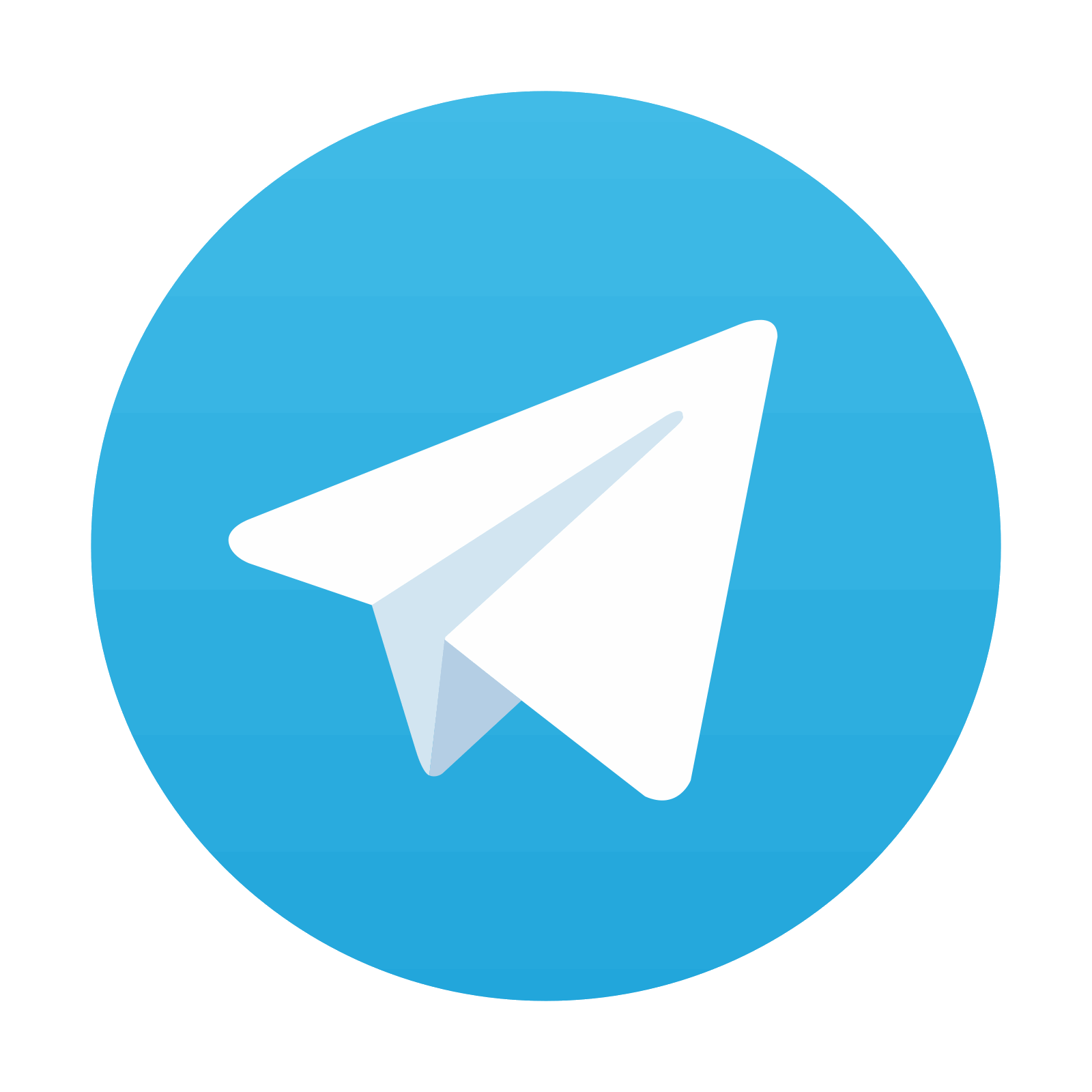
Stay updated, free dental videos. Join our Telegram channel

VIDEdental - Online dental courses
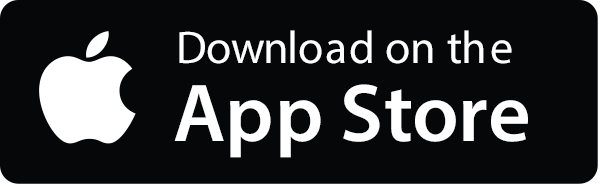
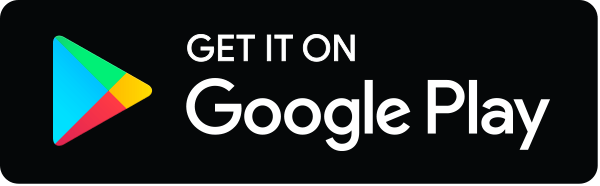