Glass–ionomer cements and resin-modified glass–ionomer cements
Introduction
Glass–ionomer cements (GICs), frequently also referred to as glass polyalkenoate cements, are restorative materials that consist of a powder and a liquid which are mixed to produce a plastic mass that subsequently sets to a rigid solid.
The GICs were first described by Wilson and Kent in 1972 and, at the time, presented a natural extension to the zinc–polycarboxylate cements that had become available in the late 1960s. The zinc–polycarboxylate cements had evolved from zinc–phosphate cement by the ingenious replacement of the phosphoric acid with polyacrylic acid (see Chapter 2.8).
The GICs were immediately seen as a potential replacement for the silicate cements that had been around for some 80 years and that were gradually being ousted by the resin-based composites.
The two main features of GICs that have allowed them to become one of the accepted dental materials are their ability to bond to enamel and dentine and their ability to release fluoride from the glass component of the cement. Thus, the GICs combine the adhesive qualities of the zinc–polycarboxylate cements with the fluoride release of the silicate cements. The relationship between the different materials is shown in Figure 2.3.1.
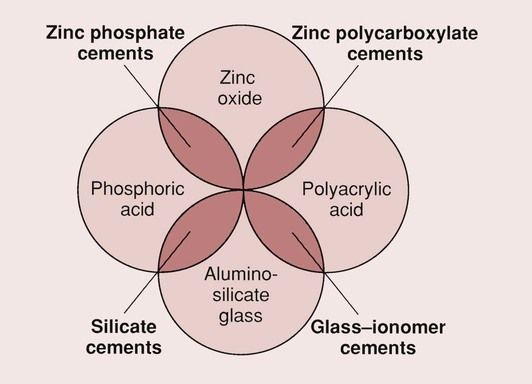
GICs were used mainly for the restoration of abrasion/erosion lesions and as a luting agent for crown and bridge reconstruction. Their clinical application has now been extended to include the restoration of proximal lesions, occlusal restorations in the deciduous dentition, cavity bases and liners and core materials by the introduction of a wide variety of new formulations.
A later innovation was the modification of the GIC by incorporating a resin, which allowed the material to be set by light activation. These new materials are, not surprisingly, known as resin-modified glass–ionomer cements (RMGICs), although sometimes they are also referred to as glass–ionomer–resin hybrids. However, the preferred description is RMGICs, in part to avoid confusion with compomers.
Thus, this group of materials deserves our closest attention.
Chemistry of glass–ionomer cements
Composition
What makes the GIC such an interesting material compared to the zinc–phosphate cements is the enormous variety of compositions that can be achieved. The main components of a GIC are glass, polyacid, water and tartaric acid.
The composition of the glass can be varied widely, giving many different properties, and, to add to this, there are numerous combinations of polyacids that are suitable for copolymerization. In contrast, for the zinc–phosphate cements, once the composition is optimized in terms of the powder-to-liquid ratio and the concentration of the phosphoric acid, there is little scope for improvement.
Of course, such a variety can be as much of a hindrance as a help, and this is reflected in the development of the GICs, which began in the early 1970s.
It could never be claimed that the GICs have had a smooth passage since their inception. The proof of this statement is based on the observation that the materials currently marketed are quite different from those originally made available for clinical use. The early materials consisted of a glass powder to which a concentrated solution of a polyacrylic acid was added. ASPA (Dentsply De Trey Ltd, Weybridge, UK) was the first commercial product, and was made available in 1976.
Glass
The glasses for the GICs contain three main components: silica (SiO2) and alumina (Al2O3) mixed in a flux of calcium fluoride (CaF2), as shown in Figure 2.3.2. The composition of the glass is largely restricted to the central region of the phase diagram by the desire to have a translucent glass.
The mixture (which also contains sodium and aluminium fluorides and calcium or aluminium phosphates as additional fluxes) is fused at a high temperature, and the molten mass is then shock-cooled and finely ground to a powder before use.
The particle size of the powder is dependent on its intended application. For filling materials, the maximum particle size is 50 µm, while for the luting and lining materials it is reduced to less than 20 µm.
The rate of release of ions from the glass (which is an important factor in determining the setting characteristics, the solubility, and the release of fluoride) is a function of the type of glass employed (see below). The glass also plays a major role in the aesthetics of the restoration, as this is dependent on both the refractive index of the glass and the presence of pigments within it.
Polyacid
There are a wide range of polyacrylic acid analogues; when these are combined with variations in molecular weight and configuration, this means that a large variety of formulations are possible. The polyacids most used in current formulations are copolymers of acrylic and itaconic acid or acrylic and maleic acid (Figure 2.3.3).
A relative newcomer is a GIC based on a copolymer of vinyl phosphonic acid. This is a much stronger acid than the others used in GICs, and the composition has to be carefully controlled to produce a cement with suitable handling properties. However, it is believed to give higher long-term strength and enhanced moisture resistance.
There is an optimum acid concentration in the case of the silicate cements, but the GICs are not so dependent on this. The strength and the resistance to aqueous attack both steadily increase with polyacid concentration, so the limiting factor is the consistency of the cement paste. The viscosity of the liquid depends on both the polyacid concentration and the molecular weight, which can vary from 10 000 to 30 000, depending on the formulation selected. Tartaric acid is an important component of the GIC, as it has a significant influence on the working and setting times.
Presentation
Powder–liquid
Many GICs consist of a glass powder to which a proprietary liquid is added. The powder is much as described above, and the liquid is an aqueous solution of polyacrylic or polymaleic acid and tartaric acid. A number of deficiencies were soon recognized with this mode of presentation and this brought about a change in formulation.
One of the problems is the excessive solubility of the cement in saliva, coupled with the slow setting reaction; another is concerned with judging the correct powder-to-liquid ratio. There is a tendency to reduce the powder content of the cement in order to obtain a smooth creamy paste, but this results in a slower-setting, weaker cement that is more susceptible to dissolution (Figure 2.3.4).
Anhydrous cements
Nowadays, many GICs are of a water-hardening type, and the cement is formed by the addition of the correct amount of distilled water. The glass powder is blended with freeze-dried polyacid and tartaric acid powder.
The first product that used this approach became available commercially in 1981. The new formulations, described as the anhydrous systems, present as a powder and a liquid. The powder contains aluminosilicate glass, polyacid powder and tartaric acid, and the liquid is just distilled water.
Capsules
It is well recognized that achieving the correct powder-to-liquid ratio can still be a problem, and a vigorous mixing process is required to ensure that all the powder is incorporated in the liquid. One way in which this may be overcome is by the use of preproportioned capsules.
The contents of different capsules do not necessarily have the same constituents, so it is inadvisable to mix them. For example, to ensure the most appropriate handling and physical properties, the filling materials have much larger glass filler particles than the luting agents. Similarly, the liquids used can vary in composition to suit the particular glass formulation and to give the correct working and setting times. This is dealt with in some detail later, in relation to the application of the different formulations.
Setting reaction
The setting reaction of the GICs is via an acid–base reaction:
MO⋅SiO2glass+H2Aacid→MAsalt+SiO2silica gel+H2O

The setting process of a GIC involves three overlapping stages:
This happens because of the different rates at which the ions are released from the glass and the rate at which the salt matrix is formed (Figure 2.3.5); as is apparent from this curve, the calcium ions are more rapidly released than the aluminium ions. This is because the calcium ions are only loosely bound in the glass structure, while the aluminium ions form part of the glass network, which is more difficult to break down. It is the calcium and the aluminium ions which will eventually form the salt matrix. The sodium and fluorine ions do not take part in the setting process but combine to be released as sodium fluoride.
Dissolution
When the proprietary solution or the water is mixed with the powder, the acid goes into solution and reacts with the outer layer of the glass. This layer becomes depleted in aluminium, calcium, sodium and fluorine ions, so that only a silica gel remains (Figure 2.3.6).
The hydrogen ions that are released from the carboxyl groups on the polyacid chain diffuse to the glass, and make up for the loss of the calcium, aluminium and fluoride ions. The setting reaction for the cement is a slow process, and it takes some time for the material to stabilize; the final translucency and colour of the material are not apparent until 24 hours after placement.
Although the material appears hard after its required setting time (usually 3–6 minutes, depending on whether it is a filling or a luting cement), it has still not reached its final physical and mechanical properties and will continue to set for up to 1 month.
Gelation
The initial set is due to the rapid action of the calcium ions, which, being divalent and more abundant initially, react more readily with the carboxyl groups of the acid than do the trivalent aluminium ions (Figure 2.3.7). This is the gelation phase of the setting reaction. The efficiency with which the calcium ions cross-link the polyacid molecules is not as good as it might be because they are also able to link carboxyl groups on the same molecule. A recent interesting development is the incorporation of zinc-containing fillers into GICs; the Zn2+ can also cross-link two polyacid molecules and may offer a more rapid set, although more data are needed before clinical conclusions can be drawn.
Various things can happen if the restoration is not protected from the outside environment during this critical phase. Aluminium ions may diffuse out of the material and be lost to the cement, thereby being unable to cross-link the polyacrylic acid chains. If the water is lost, the reaction cannot go to completion. In both instances, a weak material will result. Alternatively, additional moisture may be absorbed, which may be contaminated with blood or saliva, leading to compromised aesthetics, with the restoration looking exceptionally dull and white. The contaminating moisture will also weaken the material and may even cause it to crumble. Hence, it is essential that contamination by moisture and drying of the restoration are both avoided, at least during the initial period of setting when the material is at its most vulnerable.
Hardening
After the gelation phase, there is a hardening phase that can last as long as 7 days. It takes some 30 minutes for the uptake of aluminium ions to become significant, yet it is the aluminium ions that provide the final strength to the cement, as they are responsible for the introduction of the cross-links. In contrast to the calcium ions, the trivalent nature of the aluminium ions ensures that a high degree of cross-linking of the polymer molecules takes place (Figure 2.3.8).
There is a continuation of the formation of aluminium salt bridges, and water becomes bound to the silica gel, which now surrounds the residual core of each of the glass particles. Once the cement has fully reacted, the solubility is quite low. The final structure is as shown in Figure 2.3.9, and consists of glass particles, each of which is surrounded by a silica gel in a matrix of cross-linked polyacrylic acid.
Whereas normally it is desirable for glasses to resist ion release, in the case of the GICs a controlled release of the ions of calcium and aluminium is essential. The skill in choosing the correct glass and the correct formulation is to balance the various requirements of good handling characteristics, low solubility, adequate fluoride release and aesthetics.
Properties
Handling characteristics
The effects of the composition of the glass on the setting process are very pronounced and of considerable importance in determining the acceptability of the final handling characteristics of the cement. The Al : Si ratio of the glass for the GICs is higher than that for the silicate cements because the polyacrylic acid and its analogues are much weaker than phosphoric acid. One of the effects of this increased ratio is that the working time is reduced.
However, GICs were inclined previously to have prolonged working and setting times. This was certainly a serious problem with the earliest formulations of this cement until it was overcome by the inclusion of the optimum concentration of tartaric acid. The tartaric acid is believed to have a twofold function. First, it reacts rapidly with the calcium ions being released from the glass with the formation of calcium tartrate, which has the effect of extending the working time. This is followed by an enhancement of the rate of formation of aluminium polyacrylate cross-links, which speeds up the set (Figure 2.3.10).
By manipulation of the glass composition and particle size, and the incorporation of tartaric acid, the handling characteristics have been much improved over the years, and are now far superior to those of the first commercially available products. These improvements are shown in Table 2.3.1. As a consequence of these changes, the GICs now have a much better defined snap set.
Table 2.3.1
Handling characteristics of old and new GICs
Material | Mixing | Working | Setting | Finishing |
ASPA | 60 s | 90 s | 6 min | 24 h |
Modern GIC | 20 s | 75 s | 2 min | 7 min |
Adhesion
One of the most attractive features of GIC is that it is a bulk placement restorative material (no need for incremental placement), which is able to bond directly to dentine and enamel. It has been shown that the polyacrylate ions either react with the apatite structure (displacing calcium and phosphate ions, and creating an intermediate layer of polyacrylate, phosphate and calcium ions), or bond directly to the calcium in the apatite, as shown in Figure 2.3.11.
The bond to dentine may be a hydrogen bond type of adhesion to the collagen combined with an ionic bond to the apatite within the dentine structure. The bond strength, as measured in shear bond strength tests, would suggest that it is not particularly strong (2–7 MPa), but clinical experience would indicate that it is durable when the material is used for the restoration of erosion lesions. Whatever the details of the bonding process may be, the bond created is strong enough such that, when a GIC is debonded, the fracture will generally run through the GIC (cohesive failure) and not along the interface (adhesive failure). The major limitation on the bond strength of the GICs appears, therefore, to be its low tensile strength, which is only of the order of 7 MPa and is due to the brittle nature of these materials.
To obtain a good bond to dentine, it is advisable to treat the surface first with a dentine conditioner. The best conditioner appears to be a 20% aqueous solution of polyacrylic acid, although tannic acid has also proved to be effective. Typical tensile bond strengths that have been measured for the bond to dentine are presented in Table 2.3.2.
Table 2.3.2
Effects of surface treatments on the tensile bond strength of GICs to enamel and dentine
Surface treatment | Bond strength (MPa) |
Enamel | |
None | 3.2 |
Citric acid | 5.6 |
Polyacrylic acid | 7.1 |
Dentine | |
None | 3.1 |
Citric acid | 3.7 |
Polyacrylic acid | 6.8 |
The major purpose of the surface treatment is to remove debris and to produce a smooth, clean surface. Citric acid should not be used, as it opens up the dentinal tubules, increasing the dentine permeability and the potential for pulpal reaction. Additionally, it demineralizes the dentine, which may compromise the bond to the apatite component.
Aesthetics
A major requirement of any restorative material intended for use in anterior teeth is that it must blend in well with the surrounding tooth tissues, so as to be barely distinguishable. The factors governing this are the colour and the translucency of the restorative material.
In GICs, the colour is produced by the glass. This can be controlled by the addition/>
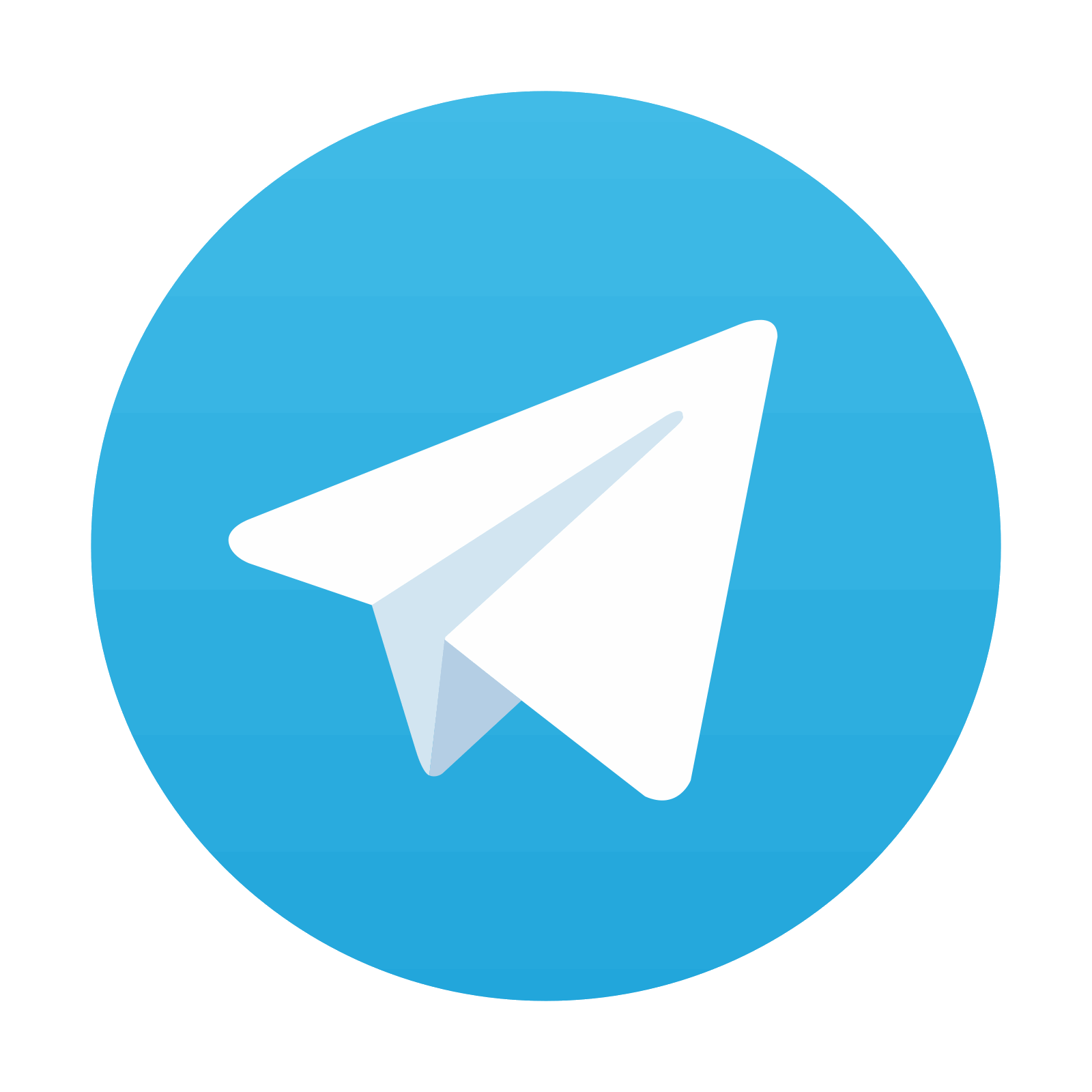
Stay updated, free dental videos. Join our Telegram channel

VIDEdental - Online dental courses
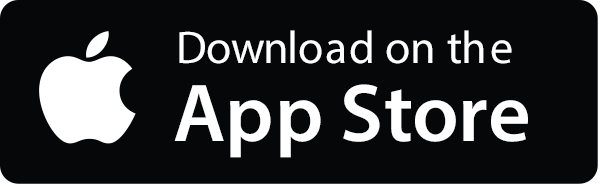
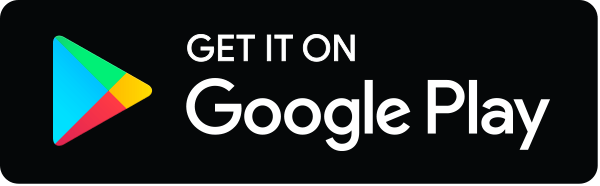