Resin composites and polyacid-modified resin composites
Introduction
A composite, as the name implies, consists of a mixture of two or more materials. Each of these materials contributes to the overall properties of the composite, and is present in its discrete form (see Chapter 1.5). Resin-based composites are possibly the most ubiquitous materials available in dentistry as they are used in a huge variety of clinical applications, ranging from filling materials, luting agents, indirect restorations and metal facings to endodontic posts and cores.
A further addition to this already extensive range of resin-based dental materials is the polyacid-modified resin composite, or compomer for short. In this chapter we will first consider the resin composites and then explore how the compomers differ from the resin composites.
Composition and structure
The resin-based composite restorative materials (‘composites’ in brief) that are used in dentistry have three major components:
The resin forms the matrix of the composite material, binding the individual filler particles together through the coupling agent (Figure 2.2.1).
Resin matrix
The resin is the chemically active component of the composite. It is initially a fluid monomer but is converted into a rigid polymer by a radical addition reaction. It is this ability to convert from a plastic mass into a rigid solid that allows this material to be used for the restoration of dentition.
The most commonly used monomer for both anterior and posterior resins is Bis-GMA, which is derived from the reaction of bisphenol-A and glycidylmethacrylate. This resin is commonly referred to as Bowen’s resin, after its inventor. It has a higher molecular weight than methyl methacrylate (MMA), which helps to reduce the polymerization shrinkage (Figure 2.2.2). The polymerization shrinkage value for MMA resins is 22 vol. %, whereas for a Bis-GMA resin it is 7.5 vol. %. There are also a number of composites that use a urethane dimethacrylate resin (UDMA) rather than Bis-GMA.
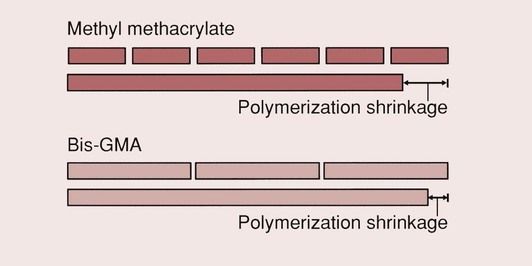
Bis-GMA and urethane dimethacrylate monomers are highly viscous fluids because of their high molecular weights; the addition of even a small amount of filler would produce a composite with a stiffness that is excessive for clinical use. To overcome this problem, low-viscosity monomers known as viscosity controllers are added, such as MMA, ethylene glycol dimethacrylate (EDMA) and triethylene glycol dimethacrylate (TEGDMA); the latter of these is most commonly used. The chemical structures of some of these monomers are presented in Figure 2.2.3.
To ensure an adequately long shelf life for the composite, it is essential that premature polymerization is prevented. To this end an inhibitor, such as hydroquinone, is included, usually in amounts of 0.1%, or less.
The resin matrix also contains the activator/initiator systems for achieving the cure. These components depend on the type of reaction employed, which may be either chemical curing or visible-light-activated curing.
Filler
A wide variety of fillers have been employed in composites to improve the properties. This practice began in the late 1950s, when fillers such as quartz were introduced into MMA-based filling materials. The inclusion of fillers offers five potentially major benefits:
The developments in filler technology lie at the root of many of the improvements that have led to the composites that are used today.
Coupling agent
In order for a composite to have acceptable mechanical properties, it is of the utmost importance that the filler and the resin are strongly bonded to each other. If there is a breakdown of this interface, the stresses developed under load will not be effectively distributed throughout the material; the interface will act as a primary source for fracture, leading to the subsequent disintegration of the composite.
The bond is achieved by the use of coupling agents that are incorporated into the resin. These coupling agents are silanes and the one most commonly used in glass-filled resin composites is γ-methacryloxypropyltriethoxysilane, or γ-MPTS for short, shown in Figure 2.2.4 (see also Chapter 1.9).
It is extremely important that there is a strong and durable bond between the resin and the filler particles. First, if there is no bond, then stress transfer between the resin and glass will be inefficient and, as a consequence, most of the stress will have to be carried by the resin matrix. This will result in excessive creep and eventually fracture and wear of the restoration. Second, the lack of bonding between the resin and the glass filler particles will create crack initiation sites. Since the resins do not have a high resistance to the propagation of cracks, this makes the composite susceptible to fatigue failure (Figure 2.2.5).
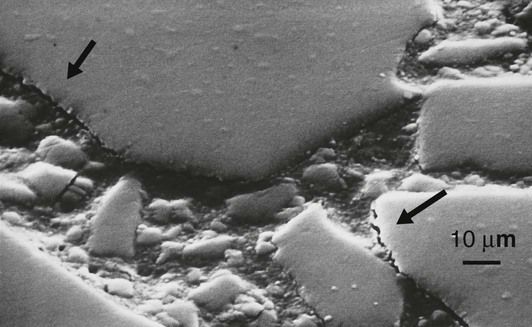
The fundamental problem is that resins are hydrophobic, whereas silica-based glasses are hydrophilic due to a surface layer of hydroxyl groups bound to the silica. Hence, the resin does not have a natural affinity to bond to the glass surface (Figure 2.2.6). The solution to the problem lies in the use of a suitable coupling agent. The silane coupling agent has been so chosen as to have hydroxyl groups on one end, which are attracted to the hydroxyl groups on the glass surface. The other end consists of a methacrylate group that is able to bond to the resin via the carbon double bond (Figure 2.2.7). A condensation reaction at the interface between the glass and the silane coupling agent ensures that the silane is covalently bonded to the glass surface (Figure 2.2.8). Improvements in the quality of the bond between the resin and the glass filler have contributed significantly to the development of wear-resistant composite restorative materials that can be used for both anterior and posterior teeth.
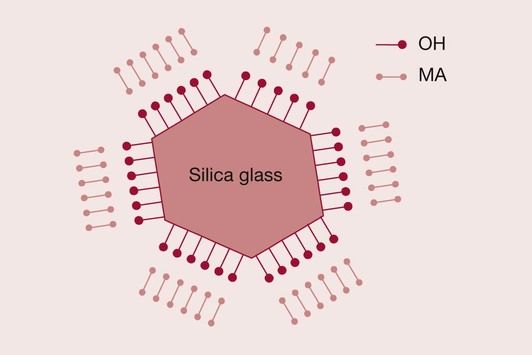
Developments in composites
A look at the changes in composites over the last couple of decades readily identifies two important areas of development:
Resin technology
Polymerization techniques
The process by which the composite paste turns into a hard material is the polymerization of the monomeric resin matrix.
With the early composites, this was achieved by supplying two pastes, a mixture of which would contain the necessary ingredients for polymerization. There would be an activator, such as a tertiary amine, in one paste, and an initiator, usually benzoyl peroxide, in the other (see Chapter 1.5 for details of this curing system).
In the early 1970s, ultraviolet (UV)-light-activated composites became available. In these materials, UV light was used to create free radicals to start the polymerization process. The energy of the UV light is sufficient to break the central bond of benzoin methyl ether to create two free radicals. Thus only a single paste was necessary, which would not set until exposed to UV light. However, there were some serious drawbacks with the use of the UV-light-cured systems. UV light can cause soft-tissue burns and can also cause damage to the eye. Hence protection needed to be used and generally great care needed to be exercised in the use of these light-curing units. The UV light source is a mercury discharge lamp, which is expensive, suffers from the problem that the intensity of the light output gradually reduces as the lamp gets older, and has a limited depth of cure due to the high degree of light absorption taking place as it travels through the composite.
Nevertheless, the practice of having a single paste, which would set hard on demand, was readily adopted by the dental profession and opened the way for the introduction of the visible-light-activated (VLA) composites. VLA composites use camphoroquinone as the source of free radicals. The energy for excitation is lower than that of benzoin methyl ether such that light with a wavelength in the blue range (~460–480 nm) is very effective. This has the advantage that a cheaper quartz halogen light source can be used and is potentially less damaging and that the light is more readily transmitted through the composite, providing greater depth of cure. Special filters are used to remove UV and infrared light for the output, so as to avoid soft-tissue burns and any excessive temperature rise respectively.
The curing methods are summarized in Figure 2.2.9.
Safety
Concern has been expressed about the safety aspects of the use of high-intensity UV light, and avoidance of these problems has been facilitated by the new VLA systems. The use of the phrase ‘visible light’ instils a feeling of safety, since it is something that we are exposed to all the time. Nevertheless, it is advisable not to expose oneself unnecessarily to the light from the visible-light production units, as high-intensity blue light can cause eye damage. The use of high-intensity light itself can have a harmful effect on the retina, and there is also the potential of damaging the retina due to the ‘blue-light hazard’. Little is known about the blue-light hazard and how serious a problem it might be. These potential problems are readily resolved by using suitable eye protection, and it is better to err on the side of caution in these matters.
Colour perception
Another difficulty that the discerning dentist needs to be aware of is that caused by a long period of exposure to high-intensity light. Such exposure can upset one’s colour perception, meaning that the selection of suitable shades of composites then becomes a real problem, especially when performing multiple restorations or when applying composite veneers.
Oxygen inhibition
Where there is an air interface with the resin, the resin will not cure and a sticky surface is readily discernible. This is of benefit when carrying out an incremental placement procedure, as it ensures that the layers of composite will be well bonded to one another. However, it can be a problem when the last increment has been placed. When it is possible to use a matrix strip, this is usually sufficient to exclude the oxygen and the resin will be fully cured up to the surface. For most resin systems, this oxygen-inhibited surface layer is very thin and extends no more than a few micrometres below the surface. Thus it is easily wiped off with a cotton pledget, e.g. when fissure sealing. However, there are some resin systems where the oxygen inhibition is considerable and a special gel needs to be used to avoid contact with oxygen in the air.
Limited depth of cure
Another reason why the VLA composites have replaced the UV systems is that the depth of cure that can be achieved with UV light is considerably less than that obtained with visible light.
In particular, there is a danger of incomplete curing with the UV systems when it is used for deep restorations, which would be a serious drawback in posterior applications. For the UV-cured composites, the maximum depth of cure is little more than 2.0 mm, while for the VLA composites a depth of cure of 3–4 mm is possible with a good light source and good technique.
Nevertheless, the depth of cure is limited for both systems, and there is always the danger that deeper parts of the restoration will not be fully cured. This is especially problematic with the proximal boxes of posterior composites (Figure 2.2.10). All can appear perfectly satisfactory on the surface, but the bases of the boxes of composite may not be fully cured, particularly when metal matrix bands are being used. A high degree of conversion of the C=C double bond in the resins is highly desirable to achieve the optimum mechanical properties and this relates to the curing time and the power of the light curing unit.
Any lack of cure provides a poor foundation for the restoration and may lead to fracture. This is due to a lack of support at the cervical margins, caused by washout of the uncured restorative material and the development of recurrent caries.
There are a number of points that need to be emphasized. The light source used with VLA composites is more accurately described as blue light rather than visible light of extremely high intensity. The typical output from a good-quality, visible-light source would produce a spectrum as shown in Figure 2.2.11. The selectivity is necessary to ensure optimal degree and depth of cure.
For all light-activated composites, the conversion from a paste to a solid material relies on the ability of the light to access and initiate the curing in all parts of the restoration. The degree to which the light can penetrate the composite is limited, so the depth to which the material can be cured is limited. A number of factors affect the depth of cure:
There is a tendency on the part of some manufacturers to recommend curing times of as little as 20 seconds, as this obviously reduces the time it takes to complete a particular procedure. This may be sufficient for applications where only a very thin layer of the composite is to be applied, but will be insufficient when adopted for extensive restorations. Curing times should be at least 40–60 seconds.
In situations where light access presents a problem, such as distal boxes of a mesial occlusal distal restoration in a posterior composite, aids to curing, such as light-conducting wedges and transparent matrices, must be considered. Curing for excessively long times is, however, not a means of getting greater depths of cure. The depth of cure for a particular composite used in conjunction with a particular light source reaches a limit, which cannot be exceeded (Figure 2.2.14). Thus, curing times of more than 60 seconds tend to be inefficient.
The interpretation of the values for the depths of cure that are quoted in the literature is fraught with difficulty. As yet, there is no recommended definition of depth of cure, and since it is highly technique-dependent, comparison of data from different sources is virtually impossible. The general rule that should be followed is that curing to a depth of greater than 2 mm should be avoided, and exposure to the light source should be for at least 40 seconds. If the cavity to be filled is deeper than 2 mm, an incremental packing technique must be employed.
Light curing units
Besides the quartz halogen light curing unit described above, there are a number of other lamps on the market. These include the blue-light-emitting diode (blue-LED), argon laser and plasma (xenon) arc lamps.
The blue-LED light curing unit has the advantage that it only emits light within a very narrow wavelength range around 460–480 nm. It is therefore ultra-energy efficient and can be operated with a small rechargeable battery, making it very mobile. However, the bandwidth of the light may be so narrow that, for some composites using a visible light curing method not incorporating camphoroquinone, its optimum light curing condition may lie outside this bandwidth. Should this be the case, then the composite will not cure or, worse still, will only cure partially, giving the impression that it has cured.
The argon laser has the advantage that it provides a very high-intensity light source, which can be optimized for the initiation of polymerization. The argon laser produces a greater depth and degree of cure in a shorter time than the halogen light curing units. This may seem very attractive at first sight, as it can significantly reduce the time of light curing by reducing the exposure times and the number of increments used in a build-up. However, the rapid cure may compromise the integrity of the resin–tooth interface, as it does not allow any stress relaxation during the curing process. It is possible that using a pulsed rather than a continuous laser can reduce this problem. One serious drawback with these curing lights is the cost, being an order of magnitude more expensive than the quartz halogen and blue-LED light curing units.
The plasma arc light curing units can deliver approximately the same high light intensity as the argon laser but at a lower cost. However, as with the argon laser, the rapid conversion of the resin can produce high shrinkage stresses and the narrow bandwidth of the light can mean that some composites will not cure.
Polymerization shrinkage
As previously noted, a long-recognized and serious drawback with composites is polymerization shrinkage. In a sense, the whole field of adhesive restorative dentistry grew from this limitation of composites, because there would invariably be a marginal gap as the composite shrinks away from the cavity wall on setting (Figure 2.2.15). Composites do not have any intrinsic defence mechanisms against caries attack, unlike glass–ionomer cements (GICs) and amalgams. Hence, once a gap is formed, micro-leakage will occur, which can quickly lead to the spread of recurrent caries.
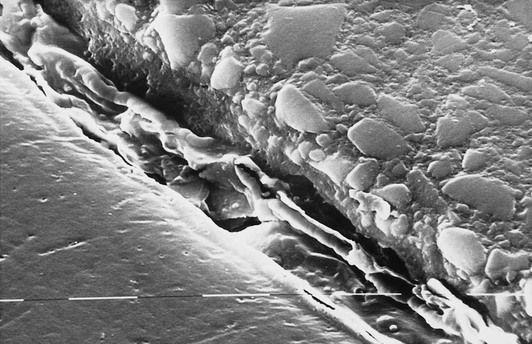
It should be noted that, while the development in light curing units has been focused on maximizing the degree of conversion of the monomer, this also maximizes the amount of polymerization shrinkage.
The polymerization shrinkage of a composite is dependent on the type of resin employed and the amount of resin present in its unpolymerized form. Most dental composites use resins with comparable polymerization shrinkage values. In general, a higher proportion of glass filler results in a lower final shrinkage. Such highly glass-filled composites do not necessarily have lower shrinkage values than the microfilled resins, as the latter use prepolymerized particles which may themselves be as highly filled as the glass particle systems.
Ideally, the polymerization shrinkage of the composite should be as low as possible, since this enhances marginal adaptation, reduces the possibility of breakdown of the bond to the tooth tissues, and inhibits the development of recurrent caries. The traditional amalgams minimize this problem because they show a slight expansion on setting, and, in due course, the gap fills with corrosion products. For high-copper-content amalgams, the shrinkage on setting is of the order of 0.1 vol. %, as compared with 2–3 vol. % for a composite. Typical values for polymerization shrinkage are shown in Figure 2.2.16. However, a note of caution is needed when examining such data, since it is difficult to find a reliable method of quantifying polymerization shrinkage and, as Figure 2.2.17 shows, a different manufacturer will tend to rank composites in a different way. Nevertheless, it is apparent that, with current resin technology, the lower limit of polymerization shrinkage is around 2.0 vol. %.
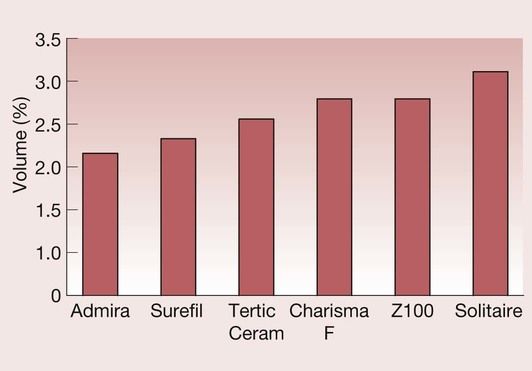
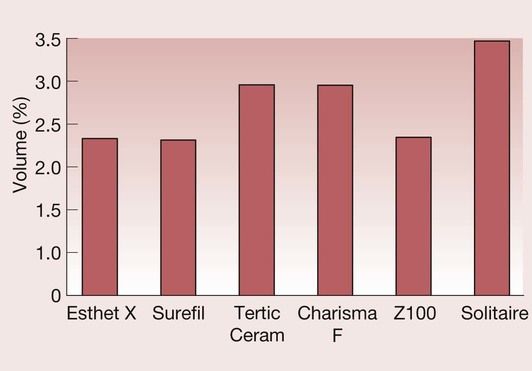
Despite major advances in the field of adhesive dental materials (see Chapter 2.5), polymerization shrinkage has been implicated as a primary source of interfacial breakdown, resulting in visible white lines or invisible cracks in the enamel and resin at the margins. The latter are only visible clinically when using transillumination and magnification. During the setting process, shrinkage stresses develop because the material is constrained by the adhesion to the cavity walls. These stresses can be sufficient to cause breakdown of the interfacial bond, whereby the advantage of the adhesive procedure is lost. This is particularly so for the bond to dentine, which is less strong than that achieved to acid-etched enamel, and, as a consequence, the shrinkage tends to occur towards the acid-etched enamel-bonded interface if the bond to the dentine breaks down (Figure 2.2.18). The gap that forms between the restoration and the dentine will give rise to postoperative sensitivity due to the hydrodynamic effect. If any of the margins are in dentine, then the breakdown of the bond will also give rise to marginal leakage. This is especially a problem when composites are placed subgingivally in proximal boxes.
Various options to overcome these problems have been proposed, which include using chemically cured composites in the base of boxes, as it is believed that the shrinkage tends to be towards the cavity walls. The use of incremental placement techniques, combined with through-the-tooth curing, is another approach that is believed will encourage polymerization shrinkage towards rather than away from the cavity walls (Figure 2.2.19).
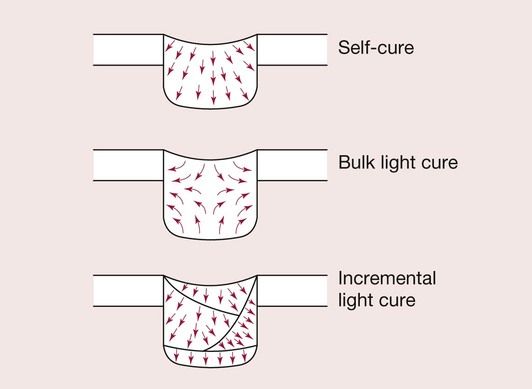
Another potential problem is that the shrinkage will cause the cusps of the tooth to be pulled inwards so that they become highly stressed. This effect has been suggested as a source of pulpal sensitivity following the placement of posterior composites. This effect can be exacerbated if a rigid, tightly bound matrix band is used during placement of a posterior composite.
It is obvious that the elimination or at least a significant reduction in the polymerization shrinkage of the resin matrix would represent a major leap forward. The steps taken to avoid or minimize the consequences of polymerization shrinkage are by far the most time-consuming aspects of the procedure for the placement of composite fillings and do not really resolve the problem satisfactorily. Ways of possibly improving the marginal integrity of composite restorations include:
Development in dentine-bonding agents continues, but there is a limit as to how much a high bond strength can compensate for polymerization shrinkage stresses and it is possible that this limit has already been reached. The idea of using a low elastic modulus liner carries with it the penalty that stresses generated by occlusal loads cannot easily be transferred across the interface between the tooth and the restoration, and may cause high stresses elsewhere in the tooth structure. The third approach is based on the idea that a reduction in the rate of reaction would allow more time for the polymerization shrinkage stresses to be dissipated by a process of flow from the free surface and stress relaxation. This has led to the introduction of a variety of soft-start blue-light curing units, using ramped, stepped and oscillating light-curing profiles (Figure 2.2.20). The clinical effectiveness of all these approaches is a matter of considerable debate in the dental research literature.
New resin technologies
Since the incorporation of glass fillers as a means of reducing polymerization shrinkage has probably gone as far as it can go (see below), the solution will most likely have to be found in the development of new resins that show little or no shrinkage on curing. A variety of different resin systems are presently being explored that include modifications of existing methacrylates such as ormocers and stress-decreasing resins and alternative chemistries such as siloranes.
Ormocers
Whereas methacrylate-based resin matrices consist of purely organic material, an alternative type of inorganic-organic copolymer resin was developed for the polymer industry more than 20 years ago and the concept adapted for use in dental resin composite restoratives. This developed into the ORMOCER®, which stands for ORganically MOdified CERamic and is a registered trademark of Fraunhofer Gesellschaft, Germany. It consists of organic reactive species with carbon double bonds for polymerization, which is bound to an inorganic Si–O–Si network (Figure 2.2.21). This inorganic-organic network exhibits a similar viscosity to Bis-GMA and thus the matrix will also contain some viscosity controllers such as TEGDMA. Admira (Voco, Cuxhaven, Germany) is a currently available ORMOCER dental restorative product.
Stress-decreasing resins
A new technology has been developed in the form of what is described as a stress-decreasing resin; it is based on the incorporation of a molecular chain that acts as a means of absorbing polymerization shrinkage stress by acting as a spring/polymerization modulator (Figure 2.2.22). Based on the scientific evidence gathered to date, the ‘polymerization modulator’ reduces stress build-up on polymerization without a reduction in the polymerization rate or conversion. It is claimed that, by use of the polymerization modulator, the resin forms a more relaxed network and provides a significantly reduced polymerization stress. A commercially available example of this new technology is a ‘Smart Dentin Replacement’ known as SDR™ from Dentsply. It should be noted that this material is primarily designed to act as a dentine substitute such that the cavity is filled up to the dentino-enamel junction with this material and then a resin composite veneer is placed over the top.
Siloranes
Unlike acrylic resins, which set by a free-radical addition polymerization, epoxy-based resins set via a quite different curing mechanism. Epoxy resin formulations harden by cationic polymerization. The term ‘epoxy’ refers to an oxygen-containing ring molecule that contains a three-membered ‘oxirane’ ring. The curing process involves ring-opening, which results in a lower net shrinkage, and the delayed consumption of reactive species can provide stress relaxation throughout polymerization, which may reduce polymerization shrinkage stress. An example of this approach that has found application in dentistry is the silorane (Figure 2.2.23), which has comparable mechanical properties to existing methacrylate composites, with reduced water sorption, improved reactivity and lower polymerization stress rates throughout curing. These novel resins also eliminate the oxygen-inhibited layer and exhibit increased ambient light stability. The material is available in a commercial form as Filtek Silorane from 3M/ESPE.
Despite the apparent reduction in polymerization shrinkage achieved in the new resin systems, it must not be as/>
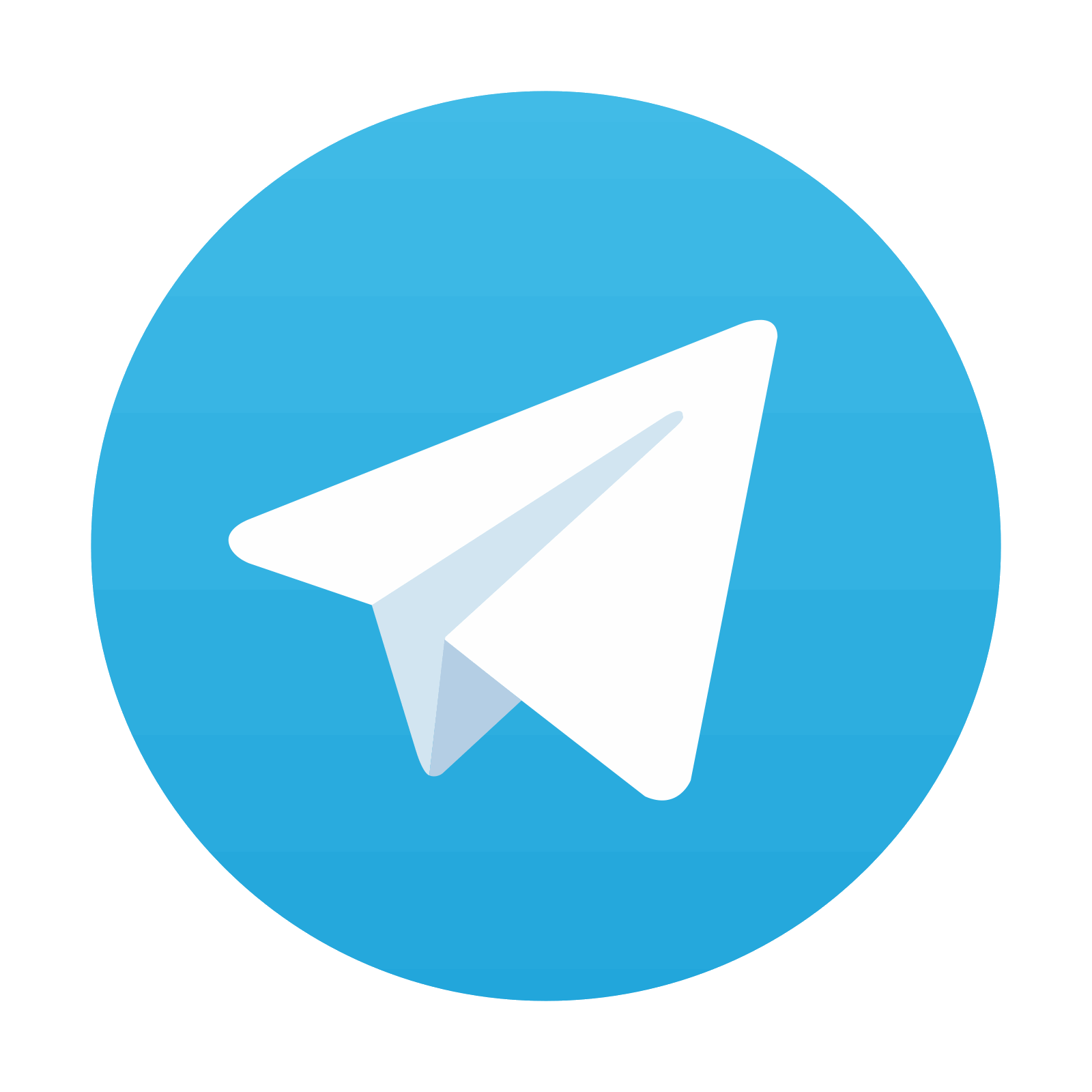
Stay updated, free dental videos. Join our Telegram channel

VIDEdental - Online dental courses
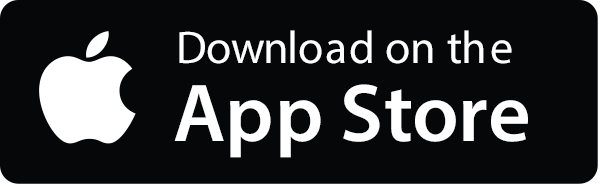
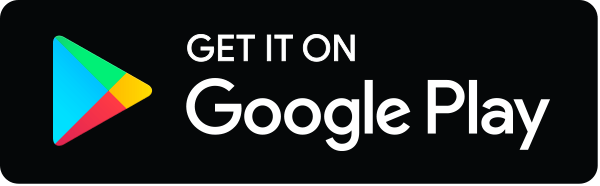