1
Contemporary Concepts of Cone Beam Computed Tomography in Orthodontics
Introduction
Truly transformative innovations are rare in most fields, but their emergence can generate a buzz that reverberates across disciplines. This is true especially in medicine and dentistry, which would stagnate without groundbreaking technologies that improve diagnosis, treatment planning, and prevention of disease. Among the advances in healthcare, radiological innovations are uniquely important as they have propelled advances in virtually most medical and dental specialties directly or indirectly. In this book, we examine how this technological cross-pollination works by detailing the broad impact of three-dimensional (3D) radiographic imaging on orthodontic diagnosis and treatment planning.
Several different technologies, including structured light, laser surface imaging, magnetic resonance imaging (MRI), computed tomography (CT), and cone beam computed tomography (CBCT), are currently available for 3D imaging. While these technologies differ in their operational details, all of them generate 3D images using the same general principles. In each of these imaging modalities, an emitted energy beam passing through or reflected from the body is modified by the structures that it encounters. A specialized sensor captures the modified energy beam, which then is converted into a 3D image by sophisticated software. Surface models, such as dental casts or slices through the 3D volume, which clearly display internal structures, can then be generated to improve diagnosis and treatment planning. Factors such as the desired image resolution, radiation exposure, soft tissue versus hard tissue visualization, and region of interest are used to determine which imaging modality is suited best for any given patient. Because of the need for orthodontists to image the craniofacial skeleton optimally and derive volumetric information, X-ray–based imaging is the best choice among these imaging technologies. Within the volumetric 3D imaging subset, CBCT, as opposed to the more expensive CT or MRI or higher radiation CT technologies, currently is the most preferred approach for such imaging.
Since the introduction of CBCT to dentistry, which first was discussed comprehensively at the 2002 symposium “Craniofacial Imaging in the 21st Century” and documented in the proceedings of the meeting (Kapila & Farman, 2003), this technology has undergone a rapid evolution and considerable integration into orthodontics (Kapila et al., 2011). Typically the pattern of integration of a new technology into a discipline, such as CBCT’s utilization in dentistry, starts with early enthusiastic adopters who hope to extend the technology’s boundaries beyond its capabilities or utility, while others wait for evidence to justify the use of such technology and still others remain skeptical that the new technology will have any impact on their modality of practice, patient care, or treatment outcomes. Given the exponentially increasing research and clinical information on CBCT, it is likely that the latter group is dwindling as more clinicians begin to recognize the usefulness of CBCT, at least for patients presenting with specific clinical challenges. On the other end of the spectrum, the routine use of CBCT on every orthodontic patient remains a controversial issue since it is not clear that the information derived from CBCT enhances diagnosis or helps in modifying treatments in several case types, which is important particularly when weighed against the risks of radiation exposure.
This varied utilization of CBCT among clinicians exists within the context of research evidence, published case reports, or anecdotal observations on topics ranging from impacted teeth to temporomandibular joint (TMJ) morphology, many of which suggest that important information indeed can be obtained through CBCT imaging. Nevertheless, scientific evidence that the utilization of CBCT alters diagnosis and improves treatment plans or outcomes has only recently begun to emerge for some of its suggested applications. Also, for several of these recommendations in which the use of CBCT is logical and/or supported by scientific evidence, the specific indications for acquisition of CBCT images and protocols for imaging and extracting appropriate information have not been resolved fully. Finally, the information obtained from CBCT imaging requires a substantial level of expertise for interpretation that orthodontists currently may not have achieved (Ahmed et al., 2012), which has attendant medico-legal implications. Thus, despite the rapidly increasing popularity of CBCT and progress in understanding and applying it to clinical orthodontics, and possibly because of the large quantities of often disparate information on this imaging technology, a cohesive, comprehensive, and objective approach to its uses and advantages in orthodontic applications currently is lacking.
This textbook provides detailed, impartial, and state-of-the-art insights, indications, protocols, procedures, innovations, and medico-legal implications of CBCT. The insights gained from CBCT are contributing to novel or refined approaches to diagnosis, treatment, and biomechanic planning (Chapters 9–23), assessment of treatment outcomes (Chapters 12–15, 17, 19–23), and providing opportunities for novel areas of research (Chapters 4, 5, 12–23). These insights have been facilitated largely by the relative advantages of CBCT imaging over radiographic two-dimensional (2D) imaging.
This chapter provides an essential overview of the topics presented in this book with the goal of highlighting the current knowledge on CBCT technology, its applications in defining 3D craniofacial anatomy and treatment outcomes, incidental findings and their medico-legal implications, and evidence-based indications and protocols for clinical applications of CBCT. In reading this chapter and book, it will become apparent that while some applications and areas have advanced sufficiently with demonstrated scientific evidence for the efficacy of CBCT in enhancing diagnosis and treatment planning, the use of CBCT in other clinical situations still is evolving. Thus, depending on where this field is in specific types of cases, the topics may range from current science to implied clinical applications to actual utility in patients who present with specific clinical findings. It is likely that as the field advances and more evidence of the efficacy of CBCT emerges, its applications in orthodontics will increase or be modified. This will enable clinicians to realize the ultimate goal of increased treatment efficiency or outcomes or both in many more clinical scenarios.
Evolution in and Basics of CBCT Technology
CBCT technology owes its inception to the discovery of X-rays by the physicist Wilhelm Conrad Röntgen in 1895, which enabled the first ever non-invasive visualization inside the human body. The discovery of X-rays was a landmark achievement in the medical field and contributed to innovative changes in how medicine and surgery are practiced. Since its initial discovery, radiographic imaging has found widespread applications in many healthcare fields. Although the images derived from the original planar X-ray technology have proven to be valuable diagnostically, they are 2D images of 3D objects, which have inherent caveats and considerable loss of information that could be of value in clinical practice or in research discoveries. Other limitations of 2D radiographic imaging include magnification, geometric distortion, superimposition of structures, projective displacements (which may elongate or foreshorten an object’s perceived dimensions), rotational errors, and linear projective transformation (Tsao et al., 1983; Quintero et al., 1999; Adams et al., 2004).
The subsequent exponential advances in computer hardware and software technologies and electrical engineering resulted in the next significant breakthrough in radiography, namely, the development of CT independently by Hounsfield and Cormack in the early 1970s (Raju, 1999; Oransky, 2004). This technological advancement enabled the generation of 3D images and the ability to view an object in its entirety from all possible viewpoints. The advantages of CT relative to 2D radiography resulted in its rapid adoption in many medical and dental fields. Successive enhancements in CT technology attributable to improvements in hardware have resulted in units with faster scanning times and relatively high image quality. In the two decades following the introduction of CT, the spiral or helical CT in effect became the standard instrument for medical imaging, which was supplanted by the multislice CT (MSCT) or multirow detector CT (MDCT) in 1998. Although medical CT has been used for craniofacial imaging from its earliest days, its utilization for this purpose increased only when high-resolution scanners with slice thicknesses of 2 mm were developed in the 1980s (Mozzo et al., 1998). However, due to the high levels of radiation, cost of the imaging units, and inaccessibility, use of medical CT for craniofacial imaging generally has been limited to patients for whom the risk-benefit ratio was considered favorable, such as for those with craniofacial anomalies, trauma, or cancer.
CBCT scanners were developed for craniofacial imaging in the late 1990s, in part to overcome several of the limitations of MSCT. In 2001, the Food and Drug Administration (FDA) approved CBCT scanners for sale in the United States, which led to their introduction into dentistry that year. CBCT differs from MSCT in the shape of the beam, the configuration of the detectors, and the software algorithms used to reconstruct the images (Figure 1.1; see also Chapter 2). These hardware and software modifications enable CBCT to capture images of the desired region of interest (ROI) with only one or two rotations, thereby decreasing the radiation exposure and time required to scan the patient compared with MSCT scans. Another advantage of CBCT scanners is their availability as compact and relatively inexpensive units that can be installed in private clinics, including those of general dentists, oral surgeons, and orthodontists (Vannier, 2003). The growing popularity and use of CBCT is evident by the more than 40 different CBCT units that now are available from more than 20 manufacturers (Molen, 2011; see also Chapters 2, 4, and 6). It is apparent that the development and availability of these specialized low-radiation-dose CBCT scanners for imaging craniofacial structures has driven the adoption and integration of 3D digital imaging into dentistry and increasingly is becoming an important source of 3D volumetric data supplemented with 2D multiplanar reconstructions (MPR; Figure 1.2) in clinical orthodontics.
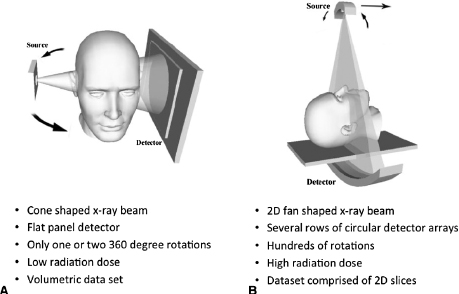
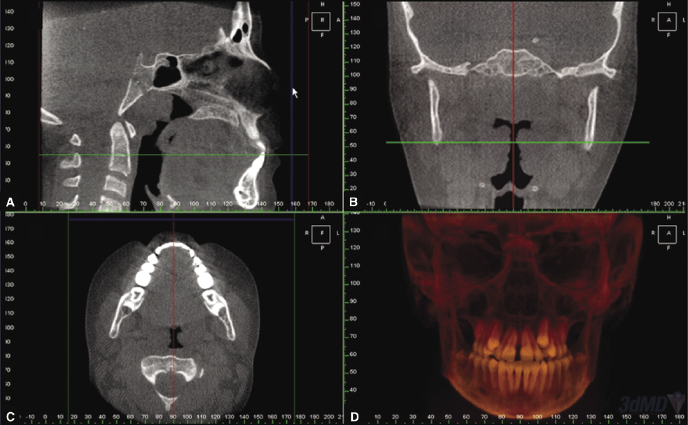
Historically, 2D imaging, including traditional radiographs and photographs, combined with 3D data obtained from models and clinic examination, has been a mainstay of orthodontic diagnosis and treatment planning. In contrast, in cases where indicated, the acquisition of clinical information entirely from 3D imaging, including CBCT, would allow for the evaluation and analysis of the true anatomy providing clinically accurate 3D representations of craniofacial structures, teeth, and roots with no superimposition of structures. Unlike several other 3D imaging methods (e.g., structured light or surface laser scanning), CBCT imaging, in addition to providing acceptable representation of soft tissue surface anatomy, has the advantage over most other 3D imaging modalities of incorporating details of underlying skeletal and dental structures, albeit with the caveat that the patient is exposed to radiation.
The pace of CBCT innovations and applications to orthodontics is reflected by the rapidly expanding numbers and quality of publications on this topic. A PubMed search using the key words CBCT or cone beam computed tomography and orthodontics generated 558 references published in English up to the end of 2013. These include three published in 2003, none in 2004, five each in 2005 and 2006, 14 in 2007, 18 in 2008, 55 in 2009, 71 in 2010, 98 in 2011, 132 in 2012, and 157 in 2013. Of these publications, a substantial subset are original or research studies that can be classified broadly into the following categories: (1) technology assessment and enhancements, (2) craniofacial and airway morphometric analyses in health and disease, (3) CBCT use in analyzing treatment outcomes, (4) incidental findings and medico-legal implications, and (5) evidence-based indications, uses, and efficacy of CBCT in diagnosis and treatment planning, all of which are discussed in greater depth in the remainder of this chapter.
Technology Assessment and Enhancements
Technology assessment studies that include radiation exposure, accuracy of measurements and images, comparison of 3D with 2D images, and advances in software and hardware technologies provide important information needed for the effective and safe utilization of CBCT.
Radiation Exposure
Radiation exposure is determined by several variables, including the type of unit used, field of exposure, pulsed versus continuous exposure, milliamperage seconds (mAs), peak kilovoltage (kVp), beam filtration, and number basis of images, several of which can be controlled by the technician or clinician. A wide variation in radiation exposure has been reported for different CBCT units (Brooks, 2009; see also Chapters 2 and 3). The field of view (FOV)-dependent effective dose of CBCT varies: 68–1074 μSv for large (>15 cm), 69–560 μSv for medium (10–15 cm), and 189–652 μSv for small (8–10 cm) FOV (Silva et al., 2008; Ludlow, 2009a, 2009b; also see Chapter 3). The effective dose for a craniofacial (large or extended) FOV CBCT scan ranges from 114 to 282 μSv when using a 10-year-old phantom and from approximately 81 to 216 μSv effective dose when using an adolescent phantom (Theodorakou et al., 2012). In contrast, although MSCT provides better soft tissue visualization than CBCT, it has a higher radiation dose of 280–1410 μSv for a maxilla-mandibular image (Loubele et al., 2005; Garcia Silva et al., 2008a; Okano et al., 2009; Suomalainen et al., 2009) and generates greater scatter from metal restorations than CBCT, which impacts the quality of the image (Farman & Scarfe, 2006; see also Chapters 2 and 3). Thus, relative to MSCT, CBCT provides appropriate levels of detail at a substantially reduced radiation exposure.
Radiation exposure is an important factor when considering whether to take a CBCT or conventional 2D radiographs. Compared with conventional 2D orthodontic radiographic series of a panoramic radiograph (2.7–24.3 μSv), a lateral cephalogram (<6 μSv) and a full-mouth series (<1.5 μSv per radiograph, or approximately 27 μSv for 18 radiographs; Garcia Silva et al., 2008a, 2008b; Ludlow et al., 2008; Ludlow & Ivanovic, 2008; Palomo et al., 2008; Okano et al., 2009), CBCT radiation exposure can be equivalent to or greater than traditional imaging depending on the FOV and age of the patient (Silva et al., 2008; SEDENTEXCT, 2011; also see Chapter 3). More specifically, when comparing radiation exposure for the large or extended FOV CBCT preferred by those clinicians who undertake CBCT in lieu of the standard orthodontic imaging, the CBCT radiation exposure derived using an adolescent or child phantom is approximately two- to ten-fold greater than the combined effective radiation dose of approximately 30 μSv from a cephalogram and panoramic radiograph (Table 1.1). The use of a dentoalveolar FOV CBCT where indicated combined with a cephalometric radiograph also has a lower effective radiation exposure than a craniofacial FOV, although this difference in radiation exposure is much less marked than when comparing the traditional 2D radiographic series with a large or extended FOV CBCT. Another approach for understanding the potential effects of radiation exposure from radiographic imaging is to compare this exposure with that from background radiation. Given that the background radiation in the United States is approximately 8 μSv per day, a large FOV would expose the patient to an equivalent of 10 to 35 days of background radiation.
Table 1.1 Comparison of effective radiation doses from conventional 2D radiography, CBCTs using pediatric phantoms for dentoalveolar (small and medium) and craniofacial (large) FOVs, MSCT, and background radiation. Most of the radiation data are provided in ranges and medians (in parentheses).
Sources of data include Loubele et al., 2005; Garcia Silva et al., 2008a, 2008b; Ludlow et al., 2008; Okano et al., 2009; Palomo et al., 2008; Theodorakou et al., 2012.
Type of radiography | Specific radiograph or methods | Effective dose (μSv) |
---|---|---|
2D radiography | Intraoral (PAs and bitewings) | 27 |
Panoramic | 2.7–24.3 | |
Cephalometric | <6 | |
Dentoalveolar FOV CBCT | 10-year-old phantom | 16–214 (43) |
Adolescent phantom | 18–70 (32) | |
Craniofacial FOV CBCT | 10-year-old phantom | 114–282 (186) |
Adolescent phantom | 81–216 (135) | |
Conventional CT | MSCT | 280–1410 |
Background radiation | 8 |
A final consideration for radiation risks, particularly for orthodontic patients, most of whom are children or adolescents, is attributable lifetime radiation risk (ICRP, 1991, 2008). This determination is based on the assumption that younger subjects are at a higher risk to the adverse effects of radiation exposure over their lifetimes than are older patients because of their length of remaining life, greater proportion of mitotic cells, and lower radiation resistance of tissues. Table 1.2 summarizes the age-related lifetime radiation risk multiplication factor based on a relative risk of one at age 30 years, which is used as the population average risk. These data show that relative to the risk of radiation exposure to a 30-year-old, children less than 10 years old have a three-fold greater radiation risk and those between 10 and 20 years have a two-fold greater attributable radiation risk; this suggests that extra caution should be exercised prior to exposing children and young adults to radiographic examination.
Table 1.2 Radiation risk in relation to age. This approach assumes a multiplicative risk projection model averaged for the two sexes. In fact, the risk for females always is higher relatively than for males.
Data are derived from ICRP (1991) and represent relative attributable lifetime risk standardized to the relative risk of 1 at age 30, which is considered the population average risk. (Reprinted with permission from SEDENTEXCT, 2009.)
Age group (years) | Multiplication factor for risk |
---|---|
<10 | ×3 |
10–20 | ×2 |
20–30 | ×1.5 |
30–50 | ×0.5 |
50–80 | ×0.3 |
80+ | Negligible risk |
Overall, irrespective of the patient’s age, it is important to weigh the risks of radiation exposure against the expected clinical benefits of imaging, given the possible sequelae of exposure to radiation (see also Chapters 3 and 6). The latter determination is based on an objective assessment of whether any additional information obtained from these scans is likely to enhance diagnosis and/or treatment planning prior to taking CBCT imaging. Conversely, it should be emphasized that radiation risks alone are not an adequate reason for not taking a CBCT scan when indicated. Instead, knowledge of radiation exposure and risks should be used to make informed decisions on when CBCT could prove to be beneficial for extracting additional diagnostic information and/or providing optimal treatment to the patient. Finally, when deciding on undertaking radiographic imaging it is important to exercise the “As Low As Reasonably Achievable” (ALARA) principle (Farman & Scarfe, 2006).
Accuracy of CBCT-Derived Cephalograms and Measurements versus Gold Standard
Studies have also been performed to determine the translatability and utility of CBCT relative to the most commonly used current methods of morphologic assessment, namely, cephalometrics and panoramic radiographs. Techniques for reconstructing cephalograms from CBCT have been developed (Farman & Scarfe, 2006) and measurements from these reconstructions can be compared directly with measurements from traditional cephalograms to assess their accuracy (Kumar et al., 2008). Such studies have revealed no significant differences in linear and angular measurements from cephalograms reconstructed from the NewTom 3G CBCT (NewTom Germany AG, Marburg, Germany) relative to conventional 2D cephalograms (Kumar et al., 2008). While these comparisons provide important information, it probably is more important to determine the accuracy of measurements from CBCT surface-rendered volumetric images to direct “gold standard” anatomical measurements made on the object of interest. Findings from studies on this subject have shown that the mean percentage measurement error for 3D CBCT is higher significantly (2.3%) than replicate skull measurements (0.6%; Periago et al., 2008). Additionally, most of the midsagittal 3D CBCT measurements were smaller systematically and significantly when using Dolphin 3D (Dolphin Imaging and Management Systems, Chatsworth, CA) software than those made directly from the skull, reflecting some potential need for image correction algorithms in the software. Similar but smaller systematic differences in 3D CBCT measurements made using NewTom HQR DVT 9000 and Hitachi MercuRay (Hitachi Medical Corp, Tokyo, Japan) versus true measurements on the skull have been reported by others (Stratemann et al., 2008). Fortunately, the majority of measurements from 3D CBCT were within 2 mm of those made directly from the skull, indicating that while the differences may be significant statistically for research purposes, they may not be relevant clinically.
As pointed out in several chapters, the direct comparison of cephalograpms with CBCT imaging may be a transitional step in the adoption of this new technology into the field. Novel approaches currently are being devised for 3D analyses and superimpositions for assessment of treatment outcomes (Chapters 19 and 21), monitoring disease progression and responses to therapy (Chapter 12), and research purposes (Chapter 4) that likely will result in the traditional 2D analyses methods becoming less relevant in specific case types and in research in orthodontics.
Comparison of CBCT versus Panoramic Radiograph
Qualitative assessments also have been made to determine whether CBCT images provide more detailed information than routine orthopantomograms or panoramic radiographs in various orthodontically relevant situations. A subjective comparison of reconstructed panoramic images from two CBCT units (NewTom 9000 and Arcadis Orbic 3D; Siemens Medical Solutions, Erlangen, Germany) with routine panoramic projection demonstrated a gain in information over conventional radiography for localizing impacted and retained teeth, the presence or absence of root resorption, cleft lip and palate (CL/P), and third molar evaluation, but not for changes in the TMJ (Korbmacher et al., 2007). Other studies have shown that CBCT provides a more accurate assessment of root parallelism, root resorption, and localization of impacted teeth than do panoramic or other 2D radiographs (Peck et al., 2007; Alqerban et al., 2009a, 2009b, 2011a, 2011b; Van Elslande et al., 2010; Bouwens et al., 2011; Durack et al., 2011; Ponder et al., 2012; Ren et al., 2012; see also later and Chapters 15 and 16).
Technology Enhancements
As described in Chapters 2, 4, 5, 20, and 21, CBCT hardware and software technologies continue to undergo rapid evolution and enhancement. Indeed, CBCT units now are available with varied configurations that include adjustable or even customizable FOVs. Other discoveries and improvements in X-ray source technologies, detectors, and post-processing of images will offer further opportunities for reduction in radiation and customization of imaging protocols. Additional developments in software include introduction of user-friendly treatment planning software and the increasing automation in 3D superimposition that will be of utility in both clinical and research applications. Progress also is being made in applying new methodologies that facilitate the merging of 3D datasets from different sources and in verifying the efficacy of these enhancements in clinical decision-making. A key extension in the utility of 3D imaging involves rapid progress in technologies such as 3D printing that increasingly are becoming available for fabrication of surgical splints and specialized orthodontic appliances that also have substantial yet untapped practical applications (see also Chapter 20).
CBCT Morphometric Analyses in Health and Disease
CBCT-based 3D craniofacial and dental morphometrics is important for defining normal and abnormal 3D anatomy of structures with a potential for longer-term utility in diagnosis and treatment planning. Much work to date on this topic has focused on quantitative and qualitative determinations of the morphology of craniofacial structures, airway, TMJ, roots, and dentoalveolar boundary conditions.
Qualitative and Quantitative Assessments of Craniofacial Morphometrics
3D imaging allows for analysis of normal size, shape, and volume of various craniofacial structures and facilitates the determination of differences in these variables between bilateral structures (Stratemann et al., 2010). Although CBCT has the potential for defining craniofacial growth changes in 3D, its sole use for this purpose is highly unlikely due to radiation concerns. To date, three main methods have been utilized for analyzing 3D anatomy and changes due to treatment in craniofacial structures. The first method extends approaches that are utilized in 2D cephalometry to derive linear and angular measurements from 3D images (Jung et al., 2009; Kim et al., 2010, 2011). This approach has the caveat of reducing 3D dimensions into 2D measurements. The second method, called Closest Point analysis (Figure 12.8 and Figure 12.9B) determines the smallest displacements between two structures but does not account for changes in shape (Cevidanes et al., 2007; Almeida et al., 2011; Motta et al., 2011). Shape correspondence (Figure 12.9B) is the third method that determines the displacement of a given landmark between two time points and represents these as either vectors or color-coded maps to depict the directionality and amount of movements, respectively (Paniagua et al., 2010; see also Chapters 12, 19, and 22). In the future, it is likely that the latter and similar approaches will replace or complement linear and angular measurements made from 3D or planar reconstructions for determining treatment changes from CBCT images.
Root Morphology, Resorption, and Angulations
Root length, form, and resorption traditionally have been assessed via periapical radiographs, while post-orthodontic root parallelism and relationships customarily are determined using panoramic radiographs. Recent studies show that CBCT provides enhanced visualization of roots, making it a valuable tool for assessing pre- or post-orthodontic root resorption and parallelism. Using true anatomic root and tooth length as a gold standard, it has been shown that CBCT is at least as good as periapical radiography for assessing root and tooth length (Lund et al., 2010; Sherrard et al., 2010). Because of its ability to generate precise images of small root defects, CBCT provides more accurate insights into root resorption as well as greater sensitivity and specificity in detecting these lesions than do panoramic or other 2D radiographs (Alqerban et al., 2009a, 2009b, 2011; Durack et al., 2011; Ponder et al., 2012; Ren et al., 2012). Also relative to CBCT, panoramic radiographs underestimate external apical root resorption (EARR; Segal et al., 2004; Dudic et al., 2009) such that in vivo root resorption is diagnosed on 43% of all teeth on panoramic radiographs compared with 69% of all teeth on the CBCT images (Dudic et al., 2009). For maxillary incisors, root resorption is not diagnosed 20% of the time with panoramic radiographs and 14% of the time with CBCT images. Finally, while 2D radiographs provide visualization of only the apex and the mesial and distal root surfaces, CBCT imaging adds insights into the effects of treatment on buccal and lingual root surfaces. This has led to the novel discovery that root loss not only is present at the root apex or is symmetrical around the root surface, but also shows a slanting pattern on surfaces adjacent to the direction of tooth movement. This finding highlights the efficacy of CBCT’s 3D rendering capacity for accurate diagnosis of both EARR and other types of root resorption.
Since root parallelism is an important goal of orthodontic treatment, its accurate determination could add valuable information in assessing the quality of treatment outcomes and, possibly, of post-treatment stability. Several studies show that panoramic radiographs generate numerous errors in the assessment of root angulation. Specifically relative to true angulations, the roots of maxillary anterior teeth often appear tipped mesially, while posterior teeth appear inclined distally, and roots of all mandibular teeth appear tipped more mesially on panoramic radiographs (McKee et al., 2002; Garcia-Figueroa et al., 2008; Owens & Johal, 2008). Recent studies comparing gold standard direct measurements on typodonts or skulls demonstrate that CBCT images, though not perfect, provide improved angular measurements relative to those derived from 2D radiographs (Peck et al., 2007; Van Elslande et al., 2010; Bouwens et al., 2011). Despite these findings, the assessment of root parallelism currently does not qualify as an indication for CBCT imaging.
Bone Quality and Quantity Assessments
Evaluating the status of the alveolar housing or boundary conditions is gaining increasing importance as a component of orthodontic patient evaluation (see also Chapter 14). For example, changes in bone height and width measurements increasingly are being utilized for assessing orthodontic treatment outcomes (Garrett et al., 2008; Kartalian et al., 2010; Tai et al., 2010a; Cattaneo et al., 2011; Corbridge et al., 2011; see also Chapter 14). Although dentoalveolar bone morphology and its relationship to underlying roots is an important component of orthodontic diagnosis and treatment, some controversy remains on the accuracy, reliability, and reproducibility of CBCT in assessing bone morphology, quantity, and quality. The accuracy of bone width dimensions depends on CBCT machine-specific settings including mAs, kVp, exposure time, and sensor size, and patient-specific variables including age, bone density, and periodontal biotype (Molen, 2010). Studies have shown that bone width particularly above a specific bone thickness threshold can be determined from CBCT with a relatively high level of reliability and reproducibility and, therefore, accuracy (Leung et al., 2010; Patcas et al., 2012). Although CBCT provides accurate assessment of alveolar bone height, caution must be exercised in evaluating fenestrations due to the high number of false positives in the determination of these defects (Leung et al., 2010; Patcas et al., 2012).
Both the quantity and quality of the cortical bone and the quality of underlying trabecular bone may be important in determining primary stability of temporary anchorage devices (TADs), which, in turn, is relevant to secondary TAD stability over the longer term (Dalstra et al., 2004; Wilmes et al., 2006; Marquezan et al., 2012; see also Chapter 18). Bone density often serves as a surrogate for bone quality and typically is measured using Hounsfield Units (HU)—a method previously devised for MSCT. HU measurements allow clinicians to assess the health of tissues. For example, alveolar bone density at a proposed dental implant site can be measured with MSCT prior to surgery to determine whether the density is sufficient to support the implant or whether a bone graft should be performed first. The HU scale assigns unit values to air, soft tissues, water, and hard tissues based on their ability to attenuate an X-ray beam. On the HU scale, air has an HU value of −1000, water is 0, and dense bone is ≥+1000. Soft tissues, which have high water content, have HU values that fall between water and dense bone. Because all conventional CT machines are calibrated to ensure that the grayscale measurements on each scan are accurate, all HU measurements taken on conventional CT-generated images can be compared directly with each other. In contrast to MSCT, because the grayscale on CBCT images is not calibrated, this technology cannot be used to measure bone density accurately; in fact, it is arbitrary, which means that tissue densities on two CBCT scans, even if taken on the same patient, cannot be measured or compared directly (Katsumata et al., 2006, 2007; Swennen & Schutyser, 2006). Thus, while a CBCT image can be used to determine if an edentulous site has sufficient alveolar bone thickness to support a dental implant, it cannot be used to determine if the bone density is sufficient to withstand the surgical placement and long-term functional stresses of implant treatment. Efforts are ongoing to develop algorithms that convert specific parameters, such as a voxel volume (VV) grayscale, derived from CBCT to quantify bone density.
TMJ Anatomy and Morphology
Although little information currently is available on the efficacy of CBCT in enhancing the diagnosis of TMJ disorders over routine radiography, CBCT has been shown to be more efficacious than conventional tomography and MRI in detecting osseous changes (Honey et al., 2007; Alkhader et al., 2010). Comparison of asymptomatic control and osteoarthritic TMJs by shape correspondence also has shown significant differences between the morphologies of healthy and degenerative condyles (Cevidanes et al., 2010a). This study also revealed a significant correlation of both pain intensity and pain duration with the variations in 3D morphology of the osteoarthritic condyles. While these findings suggest the potential utility of CBCT as a diagnostic aid in TMJ osteoarthritis (OA), it is important to understand that structural bony changes of the TMJ alone do not reveal whether or not the disease is active, and no direct correlation between TMJ morphological changes and clinical findings in OA or other arthritides exists.
Airway Morphology and Relationship to Obstructive Sleep Apnea (OSA)
As reported later in this book (see Chapter 13) and in a recent publication (Schendel & Hatcher, 2010), CBCT can be used to image the airway accurately to provide cross-sectional area, minimum cross-section, and total airway volume (Figure 1.3). Initial investigations on airway patency, function, and disorders utilizing CBCT have provided preliminary answers including dimensions of normal airway anatomy in adults (Smith, 2009), relationship of 2D to 3D measurements (Lenza et al., 2010), differences in airway morphology in obstructive sleep apnea (OSA) and non-OSA subjects (Haponik et al., 1983; Galvin et al., 1989; Avrahami & Englender, 1995; Caballero et al., 1998; Schwab et al., 2003; Yucel et al., 2005), and the effects of rapid palatal expansion (RPE) and orthognathic surgery on airway dimensions (Samman et al., 1992; Chen et al., 2007; Degerliyurt et al., 2008; Oliviera de Felippe et al., 2008; Hong et al., 2011; see also Chapters 13, 17, and 22). Several of these studies show no relationship between 2D linear and 3D cross-sectional areas of airway, which suggests that the use of 2D data may not be valid for assessing airway patency. Also up to this time, no studies have demonstrated that qualitative or quantitative assessments of CBCT are capable of predicting OSA accurately.
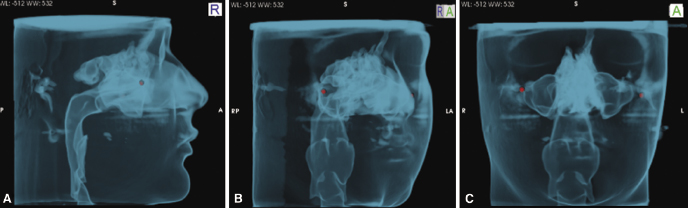
Utility of CBCT in Assessing Treatment Outcomes
Because CBCT is 3D and not subject to the error and overlap found in lateral or posterior-anterior cephalometry, it provides the capability of assessing treatment outcomes in all three planes of space as well as volumetrically. As a result of these advantages over routine radiography, CBCT is becoming an important tool in assessing outcomes of orthodontic and surgical therapies. These include the determination of the effects of treatments on orthopedic versus orthodontic changes, root resorption, bone boundary conditions, airway, alveolar grafting in CL/P, and orthognathic surgery as discussed later and in Chapters 12, 14, 15, and 17–23.
Maxillary Expansion
CBCT has enabled more in-depth dissection of responses of bone and teeth to maxillary expansion than was possible through 2D radiography or study models. For example, studies on four-banded maxillary expanders have revealed that although the first premolar, second premolar, and first molar all have similar magnitudes of total overall expansion (which includes skeletal expansion, dental tipping, and alveolar bone bending), the skeletal expansion is greater in the anterior than posterior maxilla (Rungcharassaeng et al., 2007; Garrett et al., 2008; Kartalian et al., 2010). These differences in findings between skeletal and overall expansion result from increasingly greater alveolar bone bending and the buccal crown tip going back from the first premolar to the first molar. Of the total expansion obtained in one of these studies with an adolescent sample (mean age 13.8 ± 1.7 years), 38% was orthopedic, 13% was due to alveolar bending, and 49% resulted from dental tipping (Garrett et al., 2008). These studies confirm that besides sutural expansion, RPE produces both dental and alveolar tipping, and suggest that much of post-expansion relapse probably occurs from “rebound” from the alveolar bending and dental tipping, since these two modalities of expansion are hard to retain. Additionally, the sample showed an increase in nasal width and decrease in maxillary sinus width (Garrett et al., 2008). In contrast to expansion using the fixed RPE appliance, a removable Schwarz appliance achieves expansion in both arches through alveolar buccal tipping (Tai et al., 2010a, 2010b).
Treatment Effects on Alveolar Boundary Conditions
Alveolar boundary conditions are the depth, height, and morphology of alveolar bone relative to tooth root dimensions, angulation, and spatial position (Kapila et al., 2011; see also Chapter 14). Boundary conditions are determined not only by dentoalveolar anatomy prior to treatment, but also by the bone’s adaptability during tooth movement and its morphology following the final positioning of teeth. Thus, in the context of orthodontic tooth movement, boundary conditions can be considered to be dynamic and dependent on the patient’s pre-treatment bone and gingival biotype as well as bone physiology. This implies that pre-treatment status of alveolar boundary conditions and their potential adaptation may dictate the limits of both the planned tooth movement and the final desired spatial position and angulation of the tooth.
The effect of orthodontic treatment and various appliances on bone morphology and boundary conditions in three planes of space can be assessed relatively well with CBCT, though not perfectly due to some of its technological limitations (see earlier and Chapter 14). This approach has been used to discern the potential effects of treatment- or patient-specific variables on the integrity and morphology of bone around tooth roots in full-fixed appliance therapy and with both rapid and slow expansion (Rungcharassaeng et al., 2007; Garrett et al., 2008; Kartalian et al., 2010; Tai et al., 2010a; Cattaneo et al., 2011; Corbridge et al., 2011; Johnson, 2011; Welmerink, 2012). Thus, it has been shown that routine orthodontic therapy using full-fixed appliances is accompanied by significant changes in bone width even with small amounts of buccal movement of posterior teeth (Johnson, 2011; Welmerink, 2012). This finding raises the question of whether biologically compatible expansion is possible using specific combinations of fixed appliances and wires as claimed by some manufacturers. A recent study tested such claims by evaluating the effects of active (In-Ovation R; Dentsply GAC, Islandia, NY) and passive (Damon 3 MX; Ormco Corp, Orange, CA) self-ligating brackets on alveolar boundary conditions (Cattaneo et al., 2011). Buccal cortical bone thickness on the second premolars decreased significantly with both types of appliances, even though the change in bucco-lingual tip of the teeth was the same with both systems. Thus these findings do not support the claims that specific appliances generate biologically compatible forces in which the bone remodels to maintain its integrity despite substantial arch expansion during treatment. Similarly, it also is known that buccal crown tipping during RPE is accompanied by a concomitant decrease in both buccal bone thickness and buccal marginal bone height (Rungcharassaeng et al., 2007). Finally, slow palatal expansion using quadhelix or Schwarz appliances decreases and increases, respectively, buccal and lingual bone thicknesses (Tai et al., 2010a, 2010b; Corbridge et al., 2011).
Bimaxillary protrusion in which orthodontic treatment aims to reduce the dentoalveolar prominence is an example of a patient- plus treatment-specific variable that can contribute to compromised alveolar boundary resulting in dehiscences following incisor retraction (Sarikaya et al., 2002). Similarly, post-orthodontic increase in incisal proclination is known to be a risk factor for dehiscences (Fuhrmann, 1996). Such incisor proclination-related recession may be worse in patients with thin initial symphysis bone width (Wehrbein et al., 1996; Artun & Grobety, 2001). In general, it appears that both patient- and treatment-specific variables such as pre-treatment boundary conditions, the magnitude of expected dental movements, and the potential adaptability of the bone to remodel adequately with these movements may be important to the quality and quantity of bone retained following orthodontic treatment.
Quantifying CL/P Defects and Outcomes of Alveolar Bone Grafts
Several studies have been performed using CBCT to determine its diagnostic efficacy in CL/P and to evaluate the success of alveolar bone grafts and paths of eruption of canines through grafted bone sites in these patients (Hamada et al., 2005; Oberoi et al., 2009, 2010; Quereshy et al., 2011; Garib et al., 2012; Zhang et al., 2012). In comparing CBCT with panoramic radiographs, it has been shown that while the panoramic radiograph enables the approximation of vertical bone height of the bone bridge, it does not permit determination of the buccal-palatal width of the bone, both of which can be discerned with CBCT (Hamada et al., 2005). Additionally, the CBCT images enable the visualization of the 3D morphology of the bone bridge, the relationship between the bone bridge and roots of neighboring teeth, and their periodontal condition. A subsequent study that compared patients before and after cleft grafting demonstrated an 84% bone fill of the cleft defect 1 year following the graft (Oberoi et al., 2009). This information is important in the decision process for implant placement. Additional studies have reported a large range for graft volume of between 6.1 mm3 (Oberoi et al., 2009) and 489 mm3 (Quereshy et al., 2011) at cleft sites for unilateral CL/P. Such determinations from CBCT are valuable in pre-surgical planning of graft site reconstruction and bone harvesting. In addition, studies on canine eruption following grafting demonstrate no significant differences in absolute incisal, facial, and mesial distances traveled between cleft-side and non-cleft-side canines. However, the movement of the cleft-side canines does not mimic that of the non-cleft-side canines and more of the cleft-side canines tend to be impacted. It remains to be determined whether the alveolar boundary conditions and bone quality of the bone graft contribute to these differences in canine eruption. These findings and their clinical implications are discussed in greater detail in Chapter 23.
Orthognathic Surgery
CBCT continues to provide useful information for diagnosis, treatment planning and assessment of treatment outcomes for orthognathic surgery cases, which are discussed in greater detail in Chapters 20–22. These studies include the determination of changes in 3D hard and soft tissue facial anatomy (McCance et al., 1992; Soncul & Bamber, 2004; Honrado et al., 2006; Jung et al., 2009; Baik & Kim, 2010; Lim et al., 2010; Ryckman et al., 2010; Park et al., 2012; Kim et al., 2013), maxilla-mandibular relationships and positional changes in the condyle (Magalhaes et al., 1995; Lee & Park, 2002; Baek et al., 2006, 2009; Cevidanes et al., 2007; Mucedero et al., 2008; Draenert et al., 2010; Kim et al., 2010, 2011, 2012; Jakobsone et al., 2011), post-surgical relapse (Busby et al., 2002; Cevidanes et al., 2007; Proffit et al., 2007), and airway dimensions (Cevidanes et al., 2007; Chen et al., 2007; Degerliyurt et al., 2008; Carvalho Fde et al., 2010; Almeida et al., 2011; Hong et al., 2011; Motta et al., 2011). As discussed in Chapter 21, the changes resulting from orthognathic surgery can be determined in 3D by performing a superimposition of the pre- and post-treatment scans on the anterior cranial base, enabling depiction of the magnitude and direction of treatment changes via color maps or vectors (Cevidanes et al., 2005, 2010b).
The effects of surgery on airway patency also have begun to be elucidated and generally show that post-surgical changes in the cross-sectional area of the airway vary depending on the type of surgery and the site at which the airway is being assessed (see also Chapter 22). Thus, for example, mandibular setback surgery decreases the airway sagittal cross-section at the soft palate but not at the posterior nasal spine. In contrast, while both a single-jaw mandibular setback and two-jaw maxillary advancement/mandibular setback procedure are accompanied by narrowing of the airway, the two-jaw surgery tends to demonstrate comparatively fewer negative effects on the airway (Samman et al., 1992; Chen et al., 2007; Degerliyurt et al., 2008; Hong et al., 2011). These findings have implications for selecting optimal surgical approaches for OSA patients and are pr/>
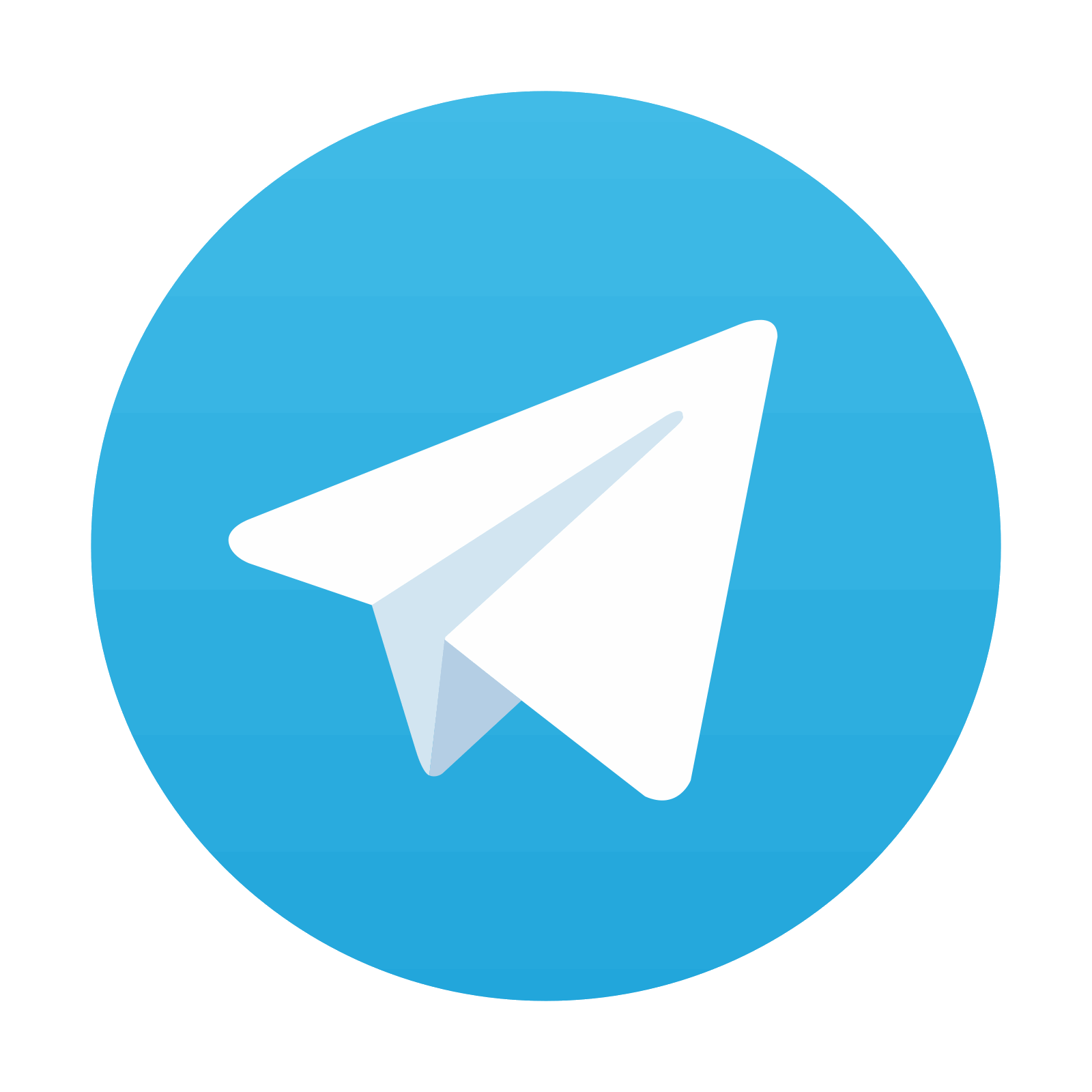
Stay updated, free dental videos. Join our Telegram channel

VIDEdental - Online dental courses
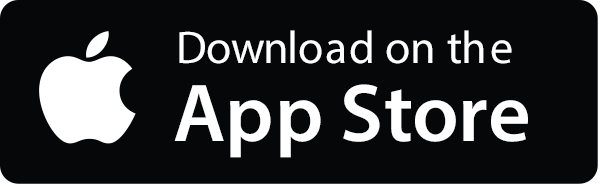
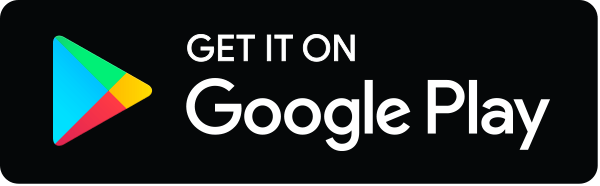