Fig. 7.1
Panoramic radiograph showing the allograft remodeling at 3 months. Note its low density similar to the native residual bone
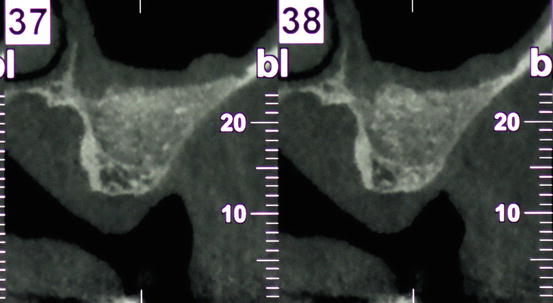
Fig. 7.2
CBCT at 5 months showing heterogeneous density of a composite particulate graft (a mixture of Mineralized Bone Allograft (Puros®, Zimmer Dental, Carlsbad, CA, USA) with mineral bovine bone (Bio-Oss®, Geistlich-Parma AG, Switzerland))
7.5.1.1 Unprocessed Allografts: Freeze-Dried Bone Allograft (FDBA)
FDBA undergoes the steps of freezing, drying, and desiccation, and it always contains its mineralized matrix, which necessitates an osteoclastic activity to liberate bone GF by the mean of “creeping substitution.”
Mineralized bone matrix has no active BMPs and therefore it lacks osteoinductive properties, although it has osteoconductive properties. In an in vitro experiment, Herold et al. (2002) claimed that the FDBA expressed less osteoblastic activity when compared to DFDBA, due to the demineralization process that released the BMPs.
On the contrary, Piatelli et al. (1996a) demonstrated that the FDBA had greater osteoinductivity than DFDBA when compared together histologically and histochemically in humans.
FDBA hardens faster than DFDBA due to its mineralization. Clinically, the residual presence of mineralized bone particles may give the impression of a more dense graft, and the longer turnover time may be of clinical benefit in larger defects in terms of gaining increased volume (Kolerman et al. 2012).
The resorption time of FDBA is extended when compared to demineralized bone, due to presence of a mineralized matrix. However, the potential advantages of the increased turnover time of the FDBA may be a disadvantage in terms of bioavailability and activity of BMPs. In theory, the mineralized component must be resorbed in order to expose the BMPs and make them biologically active. If osteoinduction is desired, one rational approach may be to combine the mineralized allograft with DFDBA or AB. With such a combination, one may take advantage of the presumed osteoinductivity and more rapid turnover time of the demineralized or AB combined with the prolonged turnover time and higher density achieved with the mineralized allograft tissue (Jensen 2006).
In a maxillary bone defect (rhesus monkey) Yukna et al. (2002) reported that the FDBA produced more novo-bone formation at a faster rate and larger quantities when compared with the DFDBA.
FDBA have been also used successfully in SFE.
It has been stated that DFDBA is less effective than FDBA in SFE (Valentini and Abensur 1997; Jensen et al. 1998) while FDBA results in more new bone growth (Cammack et al. 2005).
Clinical experience has shown that performing SFE with DFDBA resulted in the presence of a cartilaginous material after 6 months, whereas grafting with FDBA resulted in the presence of a hard, bone-like substance, which is essential when implant defects are treated, which demonstrates that the use of FDBA is more effective than DFDBA (Haas et al. 2002).
Only a limited number of human histomorphometric studies (Cammack et al. 2005) investigated the use of FDBA in SFE.
A clinical histological and histomorphometrical study (Kolerman et al. 2008) evaluated the regenerative potential of FDBA in SFE. New bone growth (29.1 %) was lower compared to the values recorded by Cammack et al. (2005) who used FDBA (41 %) or DFDBA (36 %) as BS.
This study is in agreement with Froum et al. (2006) who reported 28.3 % vital bone using a mineralized cancellous bone allograft for SFE supporting the claim that FDBA are osteoconductive BS.
7.5.1.2 Demineralized Bone Matrix (DBM; e.g., DFDBA)
DBM biologic activity is presumably attributable to the demineralization process leading to an exposure of GF (mainly BMPs) of the allograft thought to enhance the osteogenic potential of the graft material (Urist and Strates 1971).
Allogenic DBM derived from human tissues is thought to have osteoconductive and osteoinductive potential but has no osteogenic capacity because of its processing (Boyan et al. 2006). Despite DBM has demonstrated the ability to induce an osteoinductive response (for improved bone growth and fusion), the amount of BMPs (less than normal human bone) in any single allograft has shown dramatic variability (Minichetti et al. 2004; Schwartz et al. 1998), and contradictory opinions about its biological properties are still present in literature (Becker et al. 1995; Frost et al. 1982; Kübler et al. 1993; Wetzel et al. 1995; Whittaker et al. 1989). Many variables such as donor variability, particle size and shape, varying demineralizing time, percent composition of DBM powder, processing, and sterilization techniques have been considered as the consequences of the differences in the fusion rates of different commercial products (Alanay et al. 2008).
One of the major differences in processing the DBM materials is the sterilization of the allograft material to prevent the potential for disease transmission from the donor to the recipient and the graft contamination. Tissue banks take preventive measures to minimize the risk of disease transmission and contamination. The allograft tissues are treated in two steps to prevent the disease transmission and graft contamination. The first step is typically aseptic processing through chemical and physical tissues cleaning to help reduce the bioburden and the cellular antigens in the grafts. The next step is the terminal sterilization to effectively clean the allograft usually done by using irradiation or the ethylene oxide technique (Jensen 2006).
DBM does not induce any significant local immunogenic reaction, as the antigenic surface structure of the bone is destroyed during demineralization (Tuli and Singh 1978). The biological activity of DBM is most probably attributable to proteins and various GF present in the extracellular matrix and made available to the host environment by the demineralization process. Prepared by acid extraction of allografts, it retains collagen and other proteins as well as (BMPs).
The amount of BMPs in DBM is highly variable, unlike the constant low levels of GF; between and even within batches of commercially available DBM products, there are big differences (intervariability and intravariability) in BMPs (Bae et al. 2006; Wildemann et al. 2007). DBM can also be mixed with AB in the presence of large defects. A Commonly Used DBM Is Demineralized Freeze-Dried Bone (DFDBA). FDBA and DFDBA are both harvested from cadaverous sources in the same manner, with the difference being that the DFDBA material undergoes the additional step of decalcification (Mellonig et al. 1981).
The process of freeze drying reduces the antigenicity of the material (Quattlebaum et al. 1988), and the decalcification stresses the osteogenic potential by exposing BMPs, inducing host cells to differentiate into osteoblasts (Mellonig et al. 1981). DFDBA forms are processed by acid, and 40 % of the mineral content is removed leaving the organic matrix intact. This process preserves the BMPs present in the bone and therefore enhances osteoinductivity (Jensen 2006) by exposing collagen and GF such BMPs. Moreover, the collagen matrix present in DFDBA acts as a scaffold that provides osteoconductive properties alongside the osteoinductive behavior. DFDBA particles are available in a variety of sizes, and similar results have been shown with the use of particles ranging from 200 to 1,000 μm (Abubaker 1999).
Histologically, new bone formation is observed on the surface of DFDBA particles as they are simultaneously resorbed. Six to twelve months are required at least for resorption and replacement by the vital bone. Some non-vital bone particles may be observed in sites grafted with DFDBA many years after implantation (Hürzeler et al. 1997).
A long-term clinical, histologic, radiographic, and histomorphometric study (Froum et al. 1998) of the use of BS in SFE considered several factors: graft material, time allowed for graft maturation, and effect of barrier membrane placement. They found that vital bone formation in grafting procedures is time dependent. Moreover, the addition of DFDBA to the xenograft material produced a moderate increase in bone production over xenograft alone (Froum et al. 1998).
In SFE, the augmented bone height was significantly higher when DFDBA was used in comparison with the empty control group, where only a blood clot was available (Kao et al. 2012). Thick, newly formed trabecular bone was observed adjacent to the cortical bone wall of the sinus cavity. In the center of the augmented space, the particles were surrounded by fibrous connective tissue. Furthermore, the bone area was significantly higher in the small-particle DFDBA group (29 %) than in the large-particle DFDBA group (20 %) (Xu et al. 2003). In the small-particle group, newly formed bone showed many interconnections and appeared in most areas of the sinus at 8 week, while in the large-particle group, the center of the sinus cavity contained fibrous connective tissue with no evidence of ossification. Moreover, the demineralization of FDBA (DFDBA) did not yield a greater percentage of new bone formed in maxillary sinus and mandibular ridge augmentations compared to mineralized FDBA.
Overall, contradictory opinions were discussed about the real osteoinductive property of DBM (e.g., DFDBA), stating that DBM is just providing an optimal osteoconductive scaffold. DBM has also potential disadvantages such transmission of diseases that has not yet been reported but is theoretically possible (Zimmermann and Moghaddam 2011). In 1996, the Sinus Conference stated that DFDBA is not an appropriate BS because of the risk for disease transmission and pronounced resorption (Jensen et al. 1998) (Fig. 7.3).
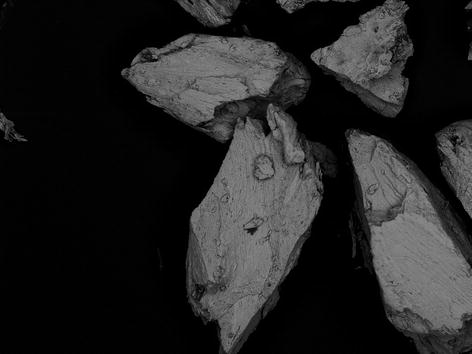
Fig. 7.3
Scanning electron microscopy (magnification ×80) of an allograft (encore®, Osteogenics, Lubbock, TX) composed of a mixture of FDBA-DFDBA (70–30)
7.5.1.3 Mineralized Bone Allograft (MBA) Cancellous or Cortical Particles (Defatted Allograft) (e.g., Puros, TBF, Biobank, etc.)
MBA is a human mineralized bone (e.g., Puros®, Biobank®, TBF®) used as an alternative allograft.
Different processing techniques for cleaning and sterilizing allografts influence the bone properties, depending on each company.
The mineralized bone allograft material is associated to type I collagen almost equal to the pristine bone. The matrix structure allows the ingrowth of vascular, cellular, and connective tissue as a critical part of de novo bone formation and remodeling. Because particle surfaces are first to interact with the host tissue, it is important to understand how various processing techniques can affect and perhaps alter the natural structure of the surface as well as the chemical composition of MBA resulting in a deleterious effect on initial healing events.
Data have demonstrated that, after processing the unwanted microbes are destroyed, but the porous bone structure is maintained, including the mineral and collagen components, in contrast to other forms of bone treatment, providing excellent bone matrix and load-bearing capabilities. The natural extracellular collagen matrix proteins are known to be important for cell attachment and bone remodeling.
In addition, animal data have confirmed that the biotolerability of the solvent-dehydrated grafts is comparable with that of cryopreserved bone grafting materials (Minichetti et al. 2004).
Although its mechanism in bone formation is still unclear, a closed-wound rabbit model experiment demonstrated that the solvent-preserved bone did not elicit a foreign body reaction and that it was the most effective graft material in inducing bone formation among the various graft materials used (Scharf 1990).
Gamma irradiation is the most commonly used form of ensuring a terminally sterilized BS. Limited-dose gamma irradiation is a key step in MBA process, since it is generally accepted that high-dose gamma sterilization (over 25 kGy) can cause damage to the collagen structure of the bone and cause denaturation of the proteins, including bone morphogenetic proteins (BMP) (Alanay et al. 2008).
In addition, this method of processing has been shown to inactivate the HIV virus and the agent responsible for Creutzfeldt-Jakob disease (Masullo C.); Taking into consideration the Estimation of the theoretical risk of transmission of Creutzfeldt-Jakob disease by human dura mater grafts manufactured by the Tutoplast® process.
Mineralized cortical bone allograft (MCBA) has biocompatible and osteoconductive potential as demonstrated in a SFE study, (Kolerman et al. 2012), permitting new bone formation (Figs. 7.4 and 7.5).
Schmitt et al. (2013) used MCBA in SFE and demonstrated comparable results to those of Froum et al. (2006) who reported averages of 28.25 and 7.65 % for vital and non-vital bone, respectively.
Other comparisons were similar (Noumbissi et al. 2005; Wood and Moore 1988). Grafting with AB or MCBA demonstrated similar outcomes; the use of MCBA tends to result in a slightly lower level of new bone formation compared to AB, but this tendency was not significant in a meta-analysis (Klijn et al. 2010a, b). MCBA can therefore be regarded as a BS that is totally resorbed and replaced by AB, integrating well into the organism (Noumbissi et al. 2005). Moreover, there is no evidence that the AB transplant is preferable regarding implant survival (Nkenke and Stelzle 2009).
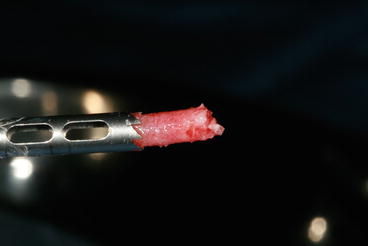
Fig. 7.4
Trephine retrieving a bone core at the implant site for histomorphometrical analysis of the bone augmentation (allograft) 5 months later: note the favorable vascularized aspect of the newly formed bone
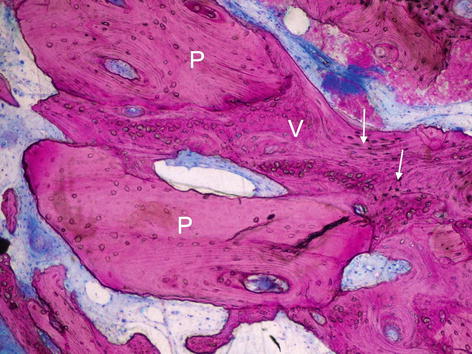
Fig. 7.5
Non-decalcified histological section showing the close contact of the newly formed bone (V) with the MCBA allograft particles (P- Puros®, Zimmer Dental, Carlsbad, CA USA). Giemsa-Paragon stain. The arrows indicate the osteocytes. P puros particles, V vital novo-bone
In conclusion, Allografts revealed the most promising results among BS in SFE.
The main advantage remains in their remodeling properties that are very similar to AB. Nevertheless, in addition to the fact that many clinicians due to their potential risk of infectious disease avoid allografts, a main concern remains regarding the maintenance of the physical dimension of the augmented bone and the long-term peri-implant bone stability.
On the other hand, any bone allograft can be enriched with growth factors (GF or cultured stromal stem cells in order to stimulate vascular invasion of the graft and new bone formation (Delloye and Bannister 2004; Delloye et al. 2004; Lucarelli et al. 2005; Schecroun and Delloye 2004).
Supplementation with these expensive biological materials appears to promote the incorporation of the bone into the host in experimental conditions, but adverse results have also been observed (Delloye and Bannister 2004). More experience with these techniques is required before they can be recommended.
7.5.2 Xenografts (e.g., Anorganic Bovine Bone)
The xenogenic grafts are taken from a donor of another species. Commonly used are anorganic bovine bone (ABB, e.g., Bio-Oss® (Geistlich Biomaterials GmbH)) and porous hydroxyapatite (pHA), derived from coral skeletons. The mineral structure and surface of ABB resembles AB. One gram of ABB has a surface of 80 m (Weibrich et al. 2000) and can therefore act as a suitable osteoconductive material (Browaeys et al. 2007). ABB contains macroscopic and microscopic structures with an interconnecting pore system that serves as a physical scaffold for the immigration of osteogenic cells (Tapety et al. 2004) (Figs. 7.6, 7.7, and 7.8). ABB osteoconductive properties, confirmed by numerous studies (Del Fabbro et al. 2008; Schlegel and Donath 1998; Schlegel 1996), derive from its chemical composition as well as macro and micromorphology. Histological studies (Froum et al. 1998; Wallace et al. 2005) revealed the presence of osteoblasts and osteoid tissue as well as apposition directly on the surface of the xenografts particles. Vital bone has been observed to bridge the gaps between xenograft particles and has been shown histologically to increase over time (Wallace et al. 1996).
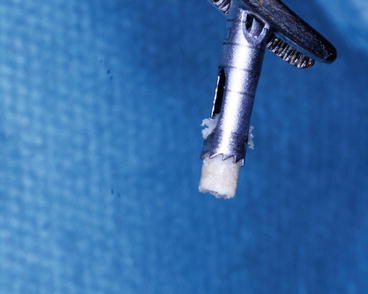
Fig. 7.6
Trephine retrieving xenograft bone core at the implant site for histomorphometrical analysis
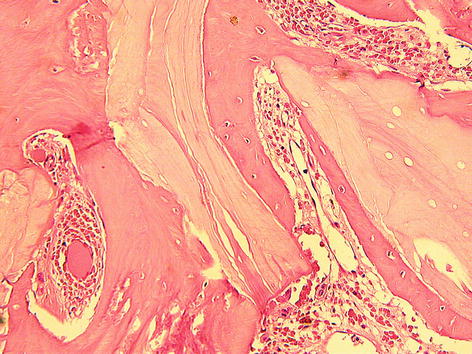
Fig. 7.7
Histologic section with hematoxylin eosin stain showing the xenograft particles (Bio-Oss®) surrounded by de novo bone
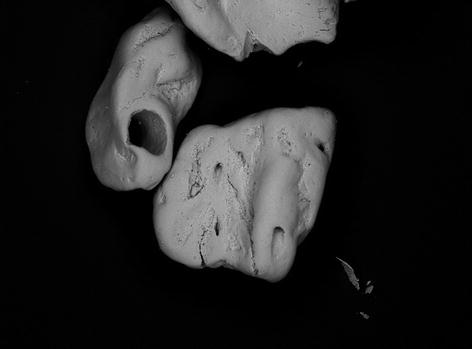
Fig. 7.8
Scanning electron microscopy (magnification ×80) of a mineral bovine bone (OCS-B®) composed of crystalline hydroxyapatite
Nevertheless, the use of nonresorbable BS results in a composite of newly formed bone and BS, not in a homogenous bone structure (Merkx et al. 2003; Petrungaro and Amar 2005).
Although this material appears to lack osteoinductive properties, it still undergoes physiologic remodeling and becomes incorporated into the bone over time (Hislop et al. 1993). Mixed clinical results with this bovine bone product have prompted some clinicians to recommend its use only as a composite graft with autogenous or allogenic bone (Gross 1997).
On the other hand, ABB appears to undergo slow or no resorption (Meijndert et al. 2005). Histologic studies based on clinical biopsies confirmed the presence of remaining ABB particles after 4.5 years (Ewers et al. 2004), 6 years (Schlegel and Donath 1998), and even 14-year post-grafting (Lezzi et al. 2007). The incomplete resorption of the ABB particles after a long period might be due to the high calcium concentration present on the biomaterial surface that could inhibit the osteoclastic resorption (Yamada et al. 1997).
Conflicting data are present in the literature about the performance of ABB over the long term, and human histologic reports are quite rare in the literature (Hämmerle et al. 1998). Resorption or a decrease in the density of the grafted area has been reported to occur over time (Sartori et al. 2003; Wallace et al. 1996), whereas other researchers found a lack of breakdown of the material (Artzi et al. 2005; Ewers et al. 2004; Merkx et al. 2003; Piattelli et al. 1999; Schlegel and Donath 1998; Skoglund et al. 1997; Valentini and Abensur 1997).
A highly purified bovine xenograft characterized by preservation of the type I collagen matrix associated with spindle-shaped hydroxyapatite crystals (Laddec® T650 Lubboc®, BioHorizons, Birmingham, USA) (Chappard et al. 1993; Poumarat and Squire 1993) has been also successfully used in SFE but showed the highest residual bone resorption 6 months when compared to AB (Papa et al. 2005).
Another study (Butz et al. 2011) demonstrated that a xenograft in the form of a putty material (PepGen P15® Putty can be used successfully for maxillary sinus augmentation.). It comprises a cell attachment peptide (P-15) that is irreversibly bound to anorganic bone matrix particles (Osteograf/N300); sodium hyaluronate (the putty) serves as a carrier material.
Referring to the authors, implant placement may be possible even after only 2 months of healing, based on histomorphometric results indicating no statistically significant difference in the newly formed bone fraction in the biopsy specimens analyzed at 2 months (21.3 % ± 2.33), at 4 months (21.9 % ± 8.9), at 6 months 28.5 % (± 6.9), and at 9 months (29.8 % ± 11.8).
Wheeler et al. (1996) found an increase in novo-bone volume from 16.38 % after 4–10 months of healing to 45.30 % after 36 months in sinus augmented with porous hydroxyapatite alone. In a clinical case report, Wallace et al. (1996) documented the sequential healing process of a sinus (4, 8, 12, and 20 months) grafted with a composite 80 % ABB (OsteoGraf) and 20 % AB. They observed slow bone formation in the sinus cavity augmented: 12–20-month period was required to convert this composite graft into a vital bone. Similar conclusions were reported by Lundgren et al. (1996) with SFE with particulated mandible and by Froum et al. (1998) with ABB.
Histological and histomorphometric studies demonstrated a better bone-to-graft contact for the ABB group compared to other BS (BCP). ABB appears to have a high osteoconductivity (Cordaro et al. 2008).
Moreover, the use of Xenografts in the sinus has been well documented in the literature. (Artzi et al. 2002; Del Fabbro et al. 2004; Hallman et al. 2002; Valentini and Abensur 2003; Wallace and Froum 2003), and numerous practitioners consider this material, alone or in combination with AB, as the BS of choice in SFE procedures. In fact, 8 published evidence-based systematic reviews concluded that the results with xenografts are the most favorable and complete (Aghaloo and Moy 2007; Del Fabbro et al. 2008; 2004; Esposito et al. 2010; Jensen and Terheyden 2009; Nkenke and Stelzle 2009; Pjetursson et al. 2008; Wallace and Froum 2003).
On the other hand, implant survival rates with ABB have been reported to be as high as or higher than those achieved with AB (Del Fabbro et al. 2008; Wallace and Froum 2003). An evidence-based review (Wallace and Froum 2003) of SFE using xenograft alone or in combination with AB or mixed with PRP showed that the survival rate of the implant placed in xenograft was statistically the same as for implant placed in particulate AB grafts. Del Fabbro et al. (2004) reported an average SR of 87.7 % of implants placed in AB, significantly lower than the 84.9 % SR of implants placed in a mixture of AB and Xenograft, and 96 % SR of implants placed in Xenograft alone. Other studies (Hallman et al. 2002; Valentini and Abensur 2003) confirmed the previous results with higher survival rates (100 %) for implants placed in sinuses using pure xenograft compared to AB or AB + xenograft.
While a main concern about AB bone resorption when used in SFE was reported at 3 years, the use of xenograft seems on the contrary to reduce the volumetric resorption as demonstrated in the Sinus Consensus Conference in 1996 (Jensen et al. 1998). Moreover, there is no evidence that this residual BS adversely affects osseointegration and, ultimately, ISR; its continual presence does not lessen the adaptive capability of the newly formed bone. The stability of the regenerated area seems to be a very important factor for the patient (Schilling et al. 2004). This is in contrast to AB, which, after 8 months of healing, have shown resorption of >50 % of the original volume (Hallman et al. 2001). It is also possible that the mechanical properties of the bone formed around the xenograft particles will improve over time in relation to bone remodeling and to the replacement of woven bone by lamellar bone (Hallman et al. 2001; Valentini et al. 1998). (Figs. 7.9, 7.10, and 7.11)
To summarize, xenografts (ABB) has been demonstrated to be resorbed very slowly (Iezzi et al. 2007; Meijndert et al. 2005; Piattelli et al. 1999); no clear evidence indicates whether this situation is an advantage or a disadvantage. As a possible advantage, this structure could represent a type of protection against bone resorption, guaranteeing the maintenance of the physical dimension and long-term stability of peri-implant bone within the augmented maxillary sinus.
However, a more recent study (Schmitt et al. 2014) evaluated the 5-year ISR after SFE with ABB and ABB plus AB with a ratio of 1/1 and found leads to a comparable amount of newly formed bone for both following SFE.
Considering that ABB is a nonresorbable BS, it can be hypothesized that this leads to stable bone over time and long-term implant success. Importantly, in the sole use of ABB, AB grafting and therefore donor site morbidities can be avoided.
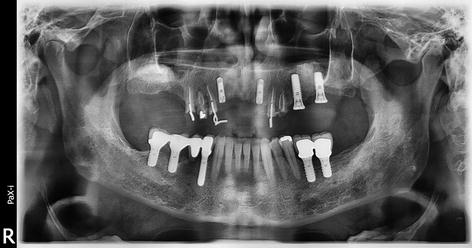
Fig. 7.9
Panoramic radiograph showing at 6 months sinus radiopacity after a bilateral SFE using a mineral bovine xenograft (Bio-Oss®, Geistlich-Parma AG, Switzerland)
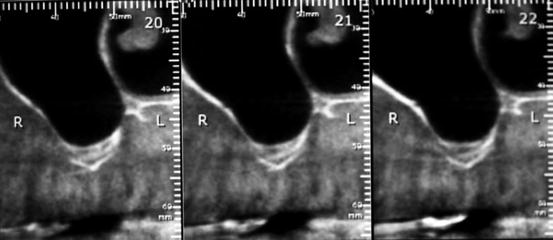
Fig. 7.10
Preoperative CBCT radiograph showing the sub-sinus residual bone volume prior to SFE
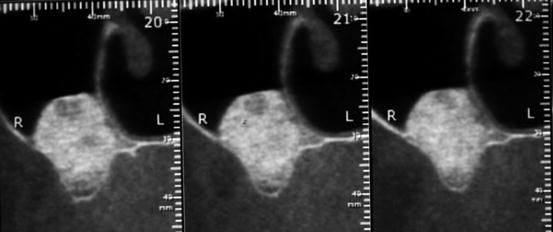
Fig. 7.11
Postoperative CBCT radiograph at 6 months showing the high density of the mineralized bovine bone (Bio-Oss, Geistlich Pharma AG, Switzerland)
7.5.3 Alloplastics (Synthetics)
Allografts and xenografts used as alternatives to AB have a potential for disease transmission (Cordioli et al. 2001). The fear of bovine spongiform encephalopathy (mad cow disease) transferring to humans (Creutzfeldt–Jakob disease) (although no report has been made in the literature) (Sogal and Tofe 1999) and the discovery of human immunodeficiency viruses surviving in allogenic bone after tissue processing have underscored concerns about disease transmission from xenografts and allografts (Marthy and Richter 1998). To what degree such BS can be considered free of prions and what are the risks of transmission of the disease to humans? Therefore, the need for safer BS has led to the development of alloplastic grafting materials.
Alloplastic materials are synthetic BS made of biocompatible, inorganic, or organic materials. They include any synthetic material not derived from a human or animal source. The main advantage of alloplasts is that they have no potential for disease transmission. They are only osteoconductive. Alloplastic materials are available in a variety of textures, sizes, and shapes. Based on their porosity, they can be classified as dense, macroporous, or microporous, and they can be either crystalline or amorphous, granular, or molded (Jensen 2006). This varying nature determines their biological features and their resorption times (Kao and Scott 2007).
However, in spite of these good properties, synthetic materials have limitations due to their poor mechanical properties and uncontrolled biodegradation in vivo (Julien et al. 2007). Therefore, surgeons have attempted to augment the activity and physical properties with composite grafts combining molecular, cellular, and genetic tissue engineering technologies (Boyne et al. 1998; Howell et al. 1997a; Margolin et al. 1998).
There are several types of alloplastic substances in clinical use nowadays: calcium phosphate-based (CaPs), other ceramics (e.g., Hydroxyapatite – HA), biphasic calcium phosphate (BCP), tricalcium phosphate (TCP), calcium sulfate, and biocompatible composite polymers.
7.5.3.1 Calcium Phosphate-Based Ceramics
The calcium phosphate family of synthetic bone grafts has both osteointegrative and osteoconductive properties. They have an excellent record of biocompatability with no reports of systemic toxicity or foreign body reactions (Hollinger and Battistone 1986).
Among different ceramic-based graft materials, hydroxyapatite (HA) and β-tricalcium phosphate (β –TCP) are the most commonly used. They are made from inorganic, nonmetallic materials with a crystalline structure, usually processed at a high temperature.
The osteoconductive CaP biomaterials allow attachment, proliferation, migration, and phenotypic expression of bone cells leading to formation of new bone in direct apposition to the CaP biomaterial. Their resorption is determined by different factors such as host and material, osteoclasts, and foreign body giant cells and should preferably go slowly. The commercial forms are granular, cement, paste, and pre-shaped wedges and shapes (Zimmermann and Moghaddam 2011).
However, CaP ceramics’ main disadvantage is their volumetric instability, which is important to facilitate bone ingrowth. Dissimilar results were found concerning the degradation of these BS (Berger et al. 1995; Daculsi 1998) as well as their biomechanical properties (Kessler et al. 2002).
In vitro dissolution of Ca–P materials depends on composition and the geometrical structural features such as particle size, porosity, surface area, shape, and crystallinity that characterize the various scaffolds.
These scaffolds should have an internal structure permissive for osseous invasion (Eggli et al. 1988; Hing et al. 2004), and their chemical composition is crucial for the osteoconductive properties of the material (Mastrogiacomo et al. 2005).
The structure of a bioceramic must possess nanopores, micropores, and macropores as these intervene in the different phases of protein adsorption, cell adhesion, and depositing of new bone over and within the BS (Fan et al. 2007; Gauthier et al. 1999).
Porosity is an important factor that influences the speed of degradability. The higher is the materials porosity, the faster is the degradability. The pore size should be ideally similar to that of spongious bone (Daculsi et al. 1988, 1990).
Calcium Hydroxyapatite (HA)
HA is a highly crystalline form of calcium phosphate, produced through a high-temperature reaction between 700 and 1,300 °C to form a highly crystalline structure. Its chemical composition is Ca10(PO4)6(OH)2. The most unique property of this material is its chemical similarity to the mineralized phase of the bone; this similarity accounts for its osteoconductive potential and excellent biocompatibility (Erbe et al. 2001; Ghosh et al. 2008).
HA has been established to be an excellent carrier of osteoinductive GF and osteogenic cell populations, which greatly adds to their future utility as bioactive delivery vehicles (Noshi et al. 2000).
Histologically, the HA-grafted sinuses exhibited a significant amount of new bone formation. The HA granules appeared integrated with the newly formed bone. Histomorphometric analysis revealed that delayed implant placement resulted in a greater amount of direct mineralized bone-to-implant contact in the augmented area than the simultaneous implant placement. The percentage of direct mineralized bone-to-implant contact was, however, more significant in the residual bone than in the augmented area (Browaeys et al. 2007).
No significant difference between HA and AB in terms of BIC was found (Haas et al. 2002), but both materials showed a significantly greater BIC than the control group, in which a sinus lift was executed without the use of autogenous bone or any biomaterial.
In a sinus study (Cosso et al. 2013), the mixture of HA and AB showed lower degree of resorption and higher dimensional stability when compared with autogenous bone graft alone.
Tricalcium Phosphate (TCP)
TCPs are porous, resorbable, biocompatible materials which have been used for two decades as synthetic bone space fillers in orthopedic and dental applications (Hak 2007) They provoke little if any inflammatory response, permit the ingrowth of cells and vessels (Wang et al. 1998), and have a direct connection with bony structure.
The chemical composition and crystallinity of the material are similar to those of the mineral phase of the bone.
The nominal composition of TCP is Ca3(PO4)2. It exists in either α- or ß-crystalline forms (Hak 2007). TCP is more quickly resorbable and less mechanically stable compared to HA. But, like HA, TCP is bioabsorbable and biocompatible.
TCP demonstrated good bone bonding behavior in the sinus. This was associated with the expression of alkaline phosphatase, collagen type I, osteocalcin, and bone sialoprotein in the newly formed bone and osteogenic mesenchyme in contact with the degrading particles. At 6 months, bone formation and matrix mineralization were still actively progressing in the tissue surrounding the β-TCP particles (Knabe et al. 2008) (Fig. 7.12).
The small-particle size and interconnected sponge-like microporosity are believed to improve osteoconductive properties and promote timely resorption concomitant with the process of remodeling (Ghosh et al. 2008; Hing et al. 2007).
Typically, it has been used in its granular porous form. Porous granules tend to migrate less than solid granules due to earlier fixation by fibrovascular ingrowth (Byrd et al. 1993). β-TCP undergoes reabsorption via dissolution and fragmentation over a 6–18 month period.
Knabe et al. (2008) compared two TCPs with different porosity grafted in the sinus. They observed a larger amount of bone formation and particle degradation in the apical area with TCP of higher porosity group and thus at the largest distance from the crestal bone compared to the group with lower porosity. Consequently, a greater porosity appears to be advantageous for enhancing bone formation and particle degradation.
Unfortunately, the replacement of β−TCP by the bone does not occur in an equitable way. That is why there is always less bone volume produced than the volume of β−TCP reabsorbed (Hollinger et al. 1996). For this reason, the clinical use of β−TCP has been as an adjunctive with other less reabsorbable bone graft substitutes or as an expander for AB graft.
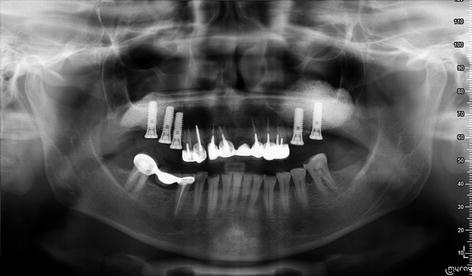
Fig. 7.12
Radiograph showing the high radiopacity of the alloplastic β-TCP Bioactive Synthetic Bone Particles (IngeniOs®) at 7 months. The grafting particles are made from silicated pure-phase beta-tricalcium phosphate
Biphasic Calcium Phosphate (BCP) (e.g., BoneCeramic®, Institut Straumann AG, Basel, Switzerland)
Although hydroxyapatite (HA)-sintered ceramics are widely used due to their osteoconductivity, its bioresorbability is so low that HA remains in the body for a long time after implantation. In contrast, tricalcium phosphate (TCP) ceramics show resorbable characters during bone regeneration and can be completely substituted for the bone tissue after stimulation of bone formation. Therefore, much attention is paid to TCP ceramics as scaffold materials for supporting bone regeneration.
The idea of mixing the HA and TCP resulted in producing BCP which is a well-known synthetic bone substitute showing biocompatibility and osteoconductive properties (Friedmann et al. 2009; Froum et al. 2008; Piattelli et al. 1996b) consisting of 60 % hydroxyapatite (HA) and 40 % beta-form of tricalcium phosphate (β−TCP), known for its osteoconductive potential that offers better bone healing conditions during the graft maturation period.
These compositions lead to partial resorption after insertion and to increased bone formation (Cordaro et al. 2008; Froum et al. 2008).
This statement was described as a possible beneficial effect on bone healing and maturation around the biomaterial particles provided by the β-TCP component of BCP during graft maturation (Cordaro et al. 2008).
Schmitt et al. (2013) showed in augmented sinuses significant less BS when using BCP compared to ABB. BCP is therefore a convenient osteoconductive BS leading to rapid bone formation at 5 months post-op. The well-known BS resorption raises the question of the long-term stability of the graft and the inserted implants. A 5-year follow-up investigation suggested the effectiveness of BCP (BoneCeramic©) in SFE, with an implant survival rate of 92.5 % after a mean follow-up of 15 months (Covani et al. 2011).
A more recent study (Piccinini et al. 2013) demonstrated the beneficial role of a composite BS, HA/TTCP (tetracalcium phosphate) in the acceleration of new bone formation, thus reducing the time needed for the graft healing in SFE.
Authors using BCP in augmented sinuses revealed a greater bone formation in areas close to the native residual bone (Avila et al. 2010; Cordaro et al. 2008; Tosta et al. 2013), while AB did not show any statistically significant differences between grafted areas far and close to the native bone. Consequently, bone formation was not predictable around the biomaterial particles in areas far from the interface with the native bone.
Similar studies also found approximately 25 % of vital bone formation in grafted sites (Artzi et al. 2005; Frenken et al. 2010; Zerbo et al. 2005). However, despite the fact that AB grafts achieved more vital bone formation in Tosta study, this had no negative clinical effect on implant outcomes, as no implant loss was observed in the BCP.
To summarize, synthetic calcium phosphate BS are widely used in SFE due to their osteoconductive and biocompatible properties. Hydroxyapatite (HA), beta-tricalcium phosphate (β-TCP), and HA/β-TCP composite are the most applied materials.
7.5.3.2 Bioactive Glasses
Bioactive glasses consist of sodium oxide, calcium oxide, phosphorus pentoxide, and silicon dioxide. Silicon dioxide (also known as silicate) forms the main component. Forms can be produced that are soluble in vivo by varying the proportions of its components.
Bioactive glasses has been shown to enhance bone repair not only by the osteoconductive properties of the particles but also by their osteo-stimulative potential defined as bone formation within internal pouches excavated within the bioglass particles away from the preexisting bony defect walls (Schepers and Ducheyne 1997).
A mechanically strong bond between bioactive glass and bone forms as a result of a silica-rich gel layer that forms on the surface of the bioactive glass when exposed to physiologic aqueous solutions. Within this gel Ca2+ and PO4 2− ions combine to form crystals of hydroxyapatite (HA) similar to that of the bone, hence a strong chemical bond (Gross et al. 1981; Hench and Wilson 1984).
Schepers and Ducheyne (1997) demonstrated bone formation in protective pouches created within the glass particles through gelation and corrosion phenomena arising from the interfacial ion exchange between the glass particles and the surrounding tissue fluids.
In a sinus study, Cordioli et al. (2001) demonstrated the presence of bands of osteoid tissue in the biopsy cores indicating that bone formation was still taking place after a healing period of 9–12 months. At that time, bioactive glass particles became smaller compared to their original size because of their partial dissolution (Schepers and Ducheyne 1997). As reported with other composite grafts (Lorenzetti et al. 1998; Wallace et al. 1996; Wheeler et al. 1996), prolonged healing periods may be required to allow bone maturation and complete resorption and substitution by bone of all bioactive glasses particles.
A variation of the bioactive glasses is the bioactive ceramics. Bioactive ceramics generally have higher strengths and improved mechanical properties over bioactive glass but both still have low fracture toughness in relation to the cortical bone (Moore et al. 2001).
Of recent interest are the bioactive glass composites, which have more elastic characteristics than the rest of the bioactive glass family. The most favorable yet is a combination of bioactive glass with a polysulfone polymer (Thompson and Hench 1998). This most closely resembles the cortical bone and is dependent on a combination of bioactivity, strength, fracture toughness, and modulus of elasticity.
To summarize, regarding recently developed alloplastic biomaterials, there is a lack of information concerning their potential to support new bone formation and maintain the gained bone volume.
Prospective studies with larger patient groups are needed to confirm the present data and also to evaluate the long-term performance of dental implants inserted in areas augmented with the most recent BS.
Some advantages of these synthetic BS are:
1.
They are osteoconductive.
2.
They have a long shelf life.
3.
There is no risk of disease/virus transfer.
4.
They are available in every shape, porosity, and composition.
5.
There is an unlimited supply.
Some disadvantages are:
1.
The handling of some synthetics is not optimal.
2.
Synthetics do not have cortical stability.
3.
They are never osteoinductive or osteogenic by themselves.
7.5.4 Adjunctives, e.g., PRP, PGRF, BMPs, Others…
Development of the bioactive surgical additives is one of the great challenges of clinical research, which has been used to regulate inflammation and increase the speed of healing process.
Several SFE studies including the addition of adjunct materials such as growth factors (GF), platelet-rich plasma (PRP), fibrin glue, or venous blood were evaluated in the literature.
Growth factors (GF) have demonstrated their efficiency in bone regeneration and can be added to all BS to promote bone regeneration. GF are present at low concentrations in bone matrix and plasma and are essential mediators of tissue repair through their stimulatory effects on angiogenesis, cell differentiation, cell proliferation, and matrix synthesis.
Numerous studies have demonstrated better results regarding the amount and rate of new bone formation when these agents were compared with traditional BS (Nevins et al. 2009; 2005).
The incorporation of GF such as transforming growth factor (TGF-βs), insulin-like growth factor (IGFs), fibroblast growth factor (FGFs), and platelet-derived growth factor (PDGFs) into the sinus graft is used as a method to reduce the healing time and enhance bone formation within the subantral environment.
The commercial availability of these growth factors (GF) has given oral and maxillofacial surgeons an additional option for the reconstruction of bony defects, but despite their potential usefulness, GF are still not available for routine use in practice.
7.5.4.1 PDGF
Among the numerous GF, recombinant human platelet-derived growth factor (rhPDGF) has received the most attention and is a well-characterized tissue GF.
PDGF is a wound-healing hormone that is naturally produced by the body at sites of soft tissue and bone injury. It has been proven to be safe and effective in a series of well-controlled human clinical trials as well as in patient use for nearly 10 years (Camelo et al. 2003; Howell et al. 1997b; Lynch et al. 1989b; Nevins et al. 2003).
The inclusion of rhPDGF in the SFE protocol used along with Bio-Oss has been associated with enhanced clinical results (Nevins et al. 2009).
7.5.4.2 Platelets-Rich Plasma (PRP)
Another GF approach is to use the patient’s own blood, separating out the platelet-rich plasma (PRP) and adding this concentrated group of autologous GFs to the grafting material (Marx et al. 1998).
Platelets are a known source of GF such as PDGF and TGF-β (Pierce et al. 1989). PRP is a platelet concentrate derived from the blood. Thus, PRP is considered to be a rich source of autologous GFs, and the contribution of PRP formulations to the bone healing process is thought to be based on the GFs contained (Harrison and Cramer 1993; Jones et al. 1992; Linder et al. 1979; Miyadera et al. 1995; Möhle et al. 1997).
Platelet gel allows access to autologous GF, which are capable of accelerating the normal processes of bone regeneration. PRP has been proposed as a useful instrument for increasing the quality and final quantity of regenerated bone in oral and maxillofacial surgery. However, the literature is conflicting with respect to the adjuvant use of PRP in SFE. The use of PRP is based on the theoretical premise that by concentrating platelets, the effects of the released growth factors (PDGF, TGF-β, IGF-I, and IGF-II) will increase. PRP growth factors do not induce osteoprogenitor cell differentiation, as is seen with the bone morphogenetic proteins, but instead act via stimulation of chemotaxis, mitogenesis, and angiogenesis of surrounding cells, acting as a catalyst in the very early phases of bone remodeling.
PRP has been used in conjunction with allografts as a source of autologous GFs (Landesberg et al. 1998), but improvement in bone formation has not been demonstrated clearly when PRP is added to BS (Sánchez et al. 2003). Another SFE study demonstrated that neither the addition of PRP to ABB (Fuerst et al. 2004) nor to AB (Jakse et al. 2003) did not show any significant improvement than the BS alone. This was expected, since PRP would act in the very early healing period, as the lifespan of a platelet in a wound and the period of the direct influence of its GF are less than 5 days (Marx et al. 1998).
The addition of PRP to ABB and AB did not improve osseointegration (Fuerst et al. 2004; Jakse et al. 2003; Roldán et al. 2004). Nevertheless, a pilot study in rabbit skull bone concluded that adding PRP to ABB is potentially beneficial (Aghaloo et al. 2004). The effect of PRP in bone graft reconstructions was demonstrated by both radiographic and histomorphometric data, which revealed more early bone formation and a higher trabecular bone density after a 6-month healing period (Marx et al. 1998).
On the contrary, other studies demonstrated that the addition of PRP to AB showed a more rapid and dense bone formation compared to AB used alone (Marx et al. 1998).
It is clear that further studies are needed to evaluate the effects of PRP on different grafting materials in SFE.
7.5.4.3 Platelet-Rich Fibrin (PRF)
Platelet-rich fibrin (PRF) is a fibrin matrix in which platelet cytokines, growth factors, and cells are trapped and may be released after a certain time and that can serve as a resorbable membrane (Naik et al. 2013). It belongs to a new generation of platelet concentrates, with simplified processing and without biochemical blood handling. Although PRF belongs to a new generation of platelet concentrates, the biologic activity of fibrin molecule is enough in itself to account for significant cicatricial capacity of the PRF. The slow polymerization mode confers to PRF membrane as a particularly favorable physiologic architecture to support the healing process. The use of platelet gel to improve bone regeneration is a recent technique in implantology. However, the biologic properties and real effects of such products remain controversial. PRF proved a slower release of growth factors than PRP and observed better healing properties with PRF (Dohan et al. 2006).
PRF was used as an additive in SFE studies. Histologic analysis in sinuses grafted using a mixture of FDBA and PRF demonstrated a reduction of healing time (to 4 months) prior to implant placement (Choukroun et al. 2006), but large-scale studies are still necessary to validate these results. Only a perfect understanding of its components and their significance will enable us to comprehend the clinical results obtained and subsequently extend the fields of therapeutic application of this protocol (Naik et al. 2013).
7.5.4.4 Bone Morphogenetic Proteins (BMPs)
The molecular approach using BMPs has received the most attention over the past decade. BMPs are differentiation factors that are members of the transforming growth factor (TGF-β) superfamily (Schmitt et al. 1999), acting as potent regulators during embryogenesis and bone and cartilage formation and repair.
A well-known GF is the bone morphogenetic protein (BMP-7 or OP-1), which is osteoinductive and may have the potential to stimulate mesenchymal cells to differentiate into bone-forming cells (Wozney et al. 1988). BMP-7 has been found to be osseoinductive and osseopromotive for osseointegration. Some studies reported the supplementation of autogenous bone-derived cells (ABC) to a cell-free grafting material such as ABB (Fuerst et al. 2004). The reason is to add AB-containing osteoblasts, combined with PRP, with the purpose of using the osteoinductive capacity of the bone.
Numerous studies reported promising results with BMPs for SFE, while others have been less enthusiastic. A human study (Boyne et al. 1997) demonstrated that recombinant BMP-2 and BMP-7 (osteogenic protein-1 [OP-1]) are each capable of stimulating bone formation in SFE. Another study in pigs suggested that rhOP-1 delivered in ABB was superior to ABB alone in SFE with simultaneous implant placement (Terheyden et al. 1999). This report concluded that there was twice the amount of bone to implant contact in the rhOP-1 group compared to the BS alone. The addition of BMP-7 to ABB resulted in significant better results compared with the combination of PRP and ABB (Roldán et al. 2004). Moreover, in SFE, BMP- 7, in addition to ABB as a growth factor, produced a significantly superior outcome, compared with ABB alone (Margolin et al. 1998; Terheyden et al. 1999).
BMP-7 in combination with an appropriate matrix has been demonstrated to be effective in accelerating osseointegration (Roldán et al. 2004). The addition of BMP-7 is dose dependent, with 2.5 mg/g collagen matrix as the most optimal concentration for inducing radiographic and histological evidence of bone formation (Margolin et al. 1998).
From a histomorphometric point of view with the BIC as parameter, the combination of ABB + BMP-7 showed the best results after 6 months. Without the addition of GF, ABB alone resulted in less BIC after 6 months (Schlegel et al. 2003).
Nevertheless, the appropriate dosage and delivery vehicles for SFE have been debated since the selected dosage of rhBMP-2 (from 1.8 to 3.4 mg) showed insufficient amounts of new bone to allow placement of dental implants; therefore, higher doses were suggested.
Moreover, a human study using rhOP-1 in SFE found that the results were too inconsistent to warrant its clinical use in SFE (Groeneveld et al. 1999).
Another SFE study investigated the bone-forming potential of rhOP-1 combined with a collagen carrier compared with AB grafts (van den Bergh et al. 2000). At 6 months, the rhOP-1 group revealed the inconsistent presence of a well-vascularized bone-like tissue. On the opposite, in the AB grafted sinuses, a bone appearance similar to the normal maxillary bone was observed histologically and clinically; implant placement was predictable at 6 months after SFE.
Based on these findings, the behavior of rhOP-1 delivered via a collagen carrier is insufficiently predictable in SFE. Further investigation is needed to demonstrate its efficiency instead of other BS.
Nowadays, BMPs are regarded as effective and safe products to enhance bone healing in SFE and will gain more and more importance in the treatment of bone defects.
However, the wide variation in results may lead to a more careful use of BMP-7 in SFE.
7.5.4.5 Hyaluronic Acid
Hyaluronic acid (HyA) is a natural polymer that has shown to be clinically advantageous due to its excellent biological properties (Lisignoli et al. 2001; Manferdini et al. 2010). In a recent study Stiller et al. (2014) mixed hyaluronic acid with β−TCP and compared this mixture with β−TCP alone in the sinus. HyA material enhanced the handling properties of the β−TCP. They concluded that both TCP grafting materials actively supported bone formation and matrix mineralization 6 months after SFE. Histomorphometric and radiologic results of TPC with HyA expressed statistically significant differences, compared to TCP alone, in terms of volume reduction, volume stability, and bone formation. Immunohistochemical osteogenic marker expression displayed also a higher tendency for TCP with HyA.
To summarize, the role of adjunctive materials in enhancing the bone graft maturation and reducing the healing time has not been completely elucidated yet. ISR in sinuses grafted with AB with adjunctive materials was 81.2 %; AB combined with allograft and adjunctive materials showed 95.3 % ISR; alloplast with adjunctive materials showed a 95.1 % ISR; and xenograft with adjunctive materials showed a 96 % ISR (Aghaloo and Moy 2007).
7.6 Graft Remodeling After the Use of Bone Substitutes
Although numerous SFE procedures have been performed to date, only a few controlled trials have addressed the healing process and the tissue changes encountered following SFE.
Graft volume stability must be considered as critical for the procedure. In fact, due to the repneumatization of the maxillary sinuses and the bone graft resorption, grafted areas may adapt considerably in shape and volume. Grafting techniques that initially only used AB showed similar results (Del Fabbro et al. 2004; Tong et al. 1998). The use of different BS has, however, introduced another variable in evaluating volume change (Kirmeier et al. 2008).
AB resorbability is a main disadvantage extensively demonstrated in numerous SFE studies (Zimmermann and Moghaddam 2011), showing significantly lower total bone volume between 4.5 and 9 months (Klijn et al. 2010a, b) due to the initial resorption of the inserted graft and the steady replacement of de novo bone (Baumgarten 2010; Consolo et al. 2007; Hallman et al. 2002; Klijn et al. 2010a, b; Lundgren et al. 1996; Pejrone et al. 2002; Simunek et al. 2008; Szabó et al. 2005; Zijderveld et al. 2005).
However, Block et al. (1998) showed that AB grafts were maintained after SFE with time and with regard to the vertical dimension, better than a combination of AB and demineralized bone.
On the other hand, intramembranous bone is more resistant to resorption than enchondral bone (Jensen and Sindet-Pedersen 1991; Kusiak et al. 1985; Zins and Whitaker 1983) due to the longer time needed for revascularization for enchondral bone grafts (Kusiak et al. 1985).
Resorption rates of up to 55 % during the first 6 months have been reported (Johansson et al. 1998) with enchondral AB, while Reinert et al. (2003) reported a vertical bone resorption of 7 % during the first year after grafting and a minimal bone loss after 12 months.
Nevertheless, no data revealed that resorption of the graft material has an influence on implant survival (Hallman and Nordin 2004; Kim et al. 2009) and should not prompt the clinician to prefer or abandon AB.
Therefore, resorption of AB and subsequent repneumatization have been mentioned as reasons to choose nonresorbable or slowly resorbable BS in SFE.
From a theorical point of view, nonresorbable BS will not remodel and functionally adapt to surrounding bone following implant placement and might be a negative mechanical factor, because it may prevent the new bone from reaching the surface of the implant. Despite the fact that the graft height is well maintained in time, it would not provide an optimal bony attachment on the implant surface (Figs. 7.3 and 7.4).
Radiological studies (Hallman et al. 2002; Hatano et al. 2004) reported a decrease in graft height 1–3 years after SFE, but subsequent changes were minimal. A significantly greater maintenance of bone height was found in intraoral grafts when compared to allografts. A combination of different BS (AB + ABB, or allograft + ABB), extensively documented lately, would be a suitable solution to promote both osteogenesis and remodeling (Hatano et al. 2004).
Bioactive glass particles, similar to composite grafts, require prolonged healing periods to allow bone maturation and complete resorption and substitution by the bone (Lorenzetti et al. 1998; Wallace et al. 1996; Wheeler et al. 1996).
In a sinus study, Cordioli et al. (2001) demonstrated the presence of bands of osteoid tissue in the biopsy cores indicating that bone formation was still taking place after a healing period of 9–12 months;
Consequently, the resorption time and the ultimate replacement of some BS with newly formed bone present a wide variation and are still not fully understood, but these BS have a relevant role in clinical practice. So the timing of implant placement should be taken in consideration when selecting a grafting material. Moreover, extended healing periods may be risky since bone volume stability may be jeopardized; therefore, implant loading may be required to limit the bone resorption, which may lead to an uncontrolled peri-implant bone loss. Below is a guide-chart table reporting healing time/loading time, based on an extensive literature review (Table 7.1).
Table 7.1
Mean healing time with different bone substitutes
Grafting material
|
Optimal healing time for implant placement following SFE (months)
|
---|---|
Autogenous bone
|
4–5
|
Allografts
|
5–6
|
Xenografts
|
7–10
|
Synthetics
|
6–12
|
7.7 Implant Survival Rate (ISR) After SFE with Different Grafting Materials
A systematic review (Del Fabbro et al. 2004) of ISR in SFE including 6,913 implants revealed an overall ISR of 91.49 %: 87.7 % when AB alone was used, 94.88 % when AB was combined with other BS, and 95.98 % when BS were used alone.
Numerous reviews demonstrated equal or better ISR with BS than those achieved with AB.
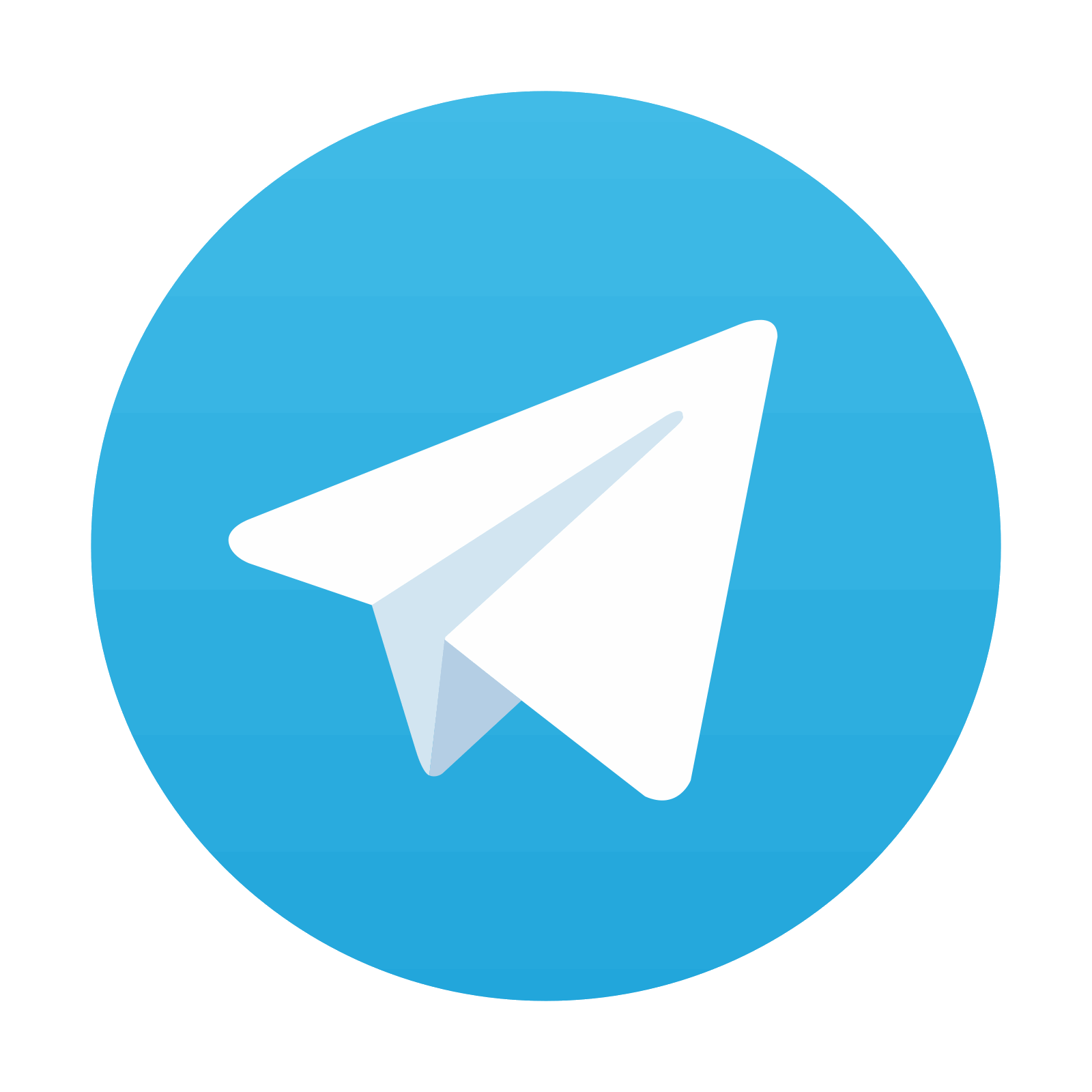
Stay updated, free dental videos. Join our Telegram channel

VIDEdental - Online dental courses
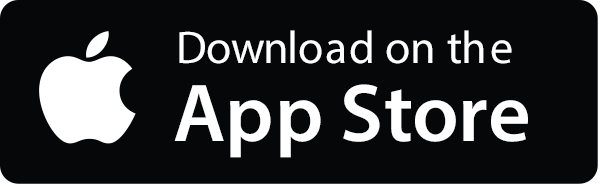
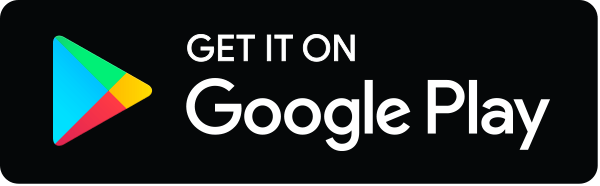