Abstract
Objectives
The aim of this study was to determine the physico-mechanical properties of a high viscosity glass ionomer cement (GIC) reinforced with TiO 2 nanotubes (TiO 2 -nt).
Methods
TiO 2 -nt was incorporated into the GIC powder components (Ketac Molar EasyMix™) in concentrations of 0% (control group), 3%, 5%, 7% by weight. Compressive strength (n = 10/group), three point bending for flexural strength (n = 18/group), microshear bond strength to dentin and failure mode (n = 20/group), and surface roughness and weight loss before and after brushing simulation (30,000 cycles) (n = 8/group) were evaluated. Data were submitted to Shapiro-Wilk, ANOVA, Tukey and Chi-square tests (α ≤ 0.05).
Results
Addition of 5% of TiO 2 -nt into GIC presented the highest values for compressive strength and differed from the control, 3% and 7% groups (p = 0.023). There were no significant differences in flexural strength (p = 0.107) and surface roughness before and after the dental brushing (p = 0.287) among the groups. GIC added with 5% TiO 2 -nt showed the lowest weight loss values (p = 0.01), whereas the control, 3% or 5% TiO 2 -nt groups presented similar microshear bond strength values (p ≥ 0.05). The 5% TiO 2 -nt group featured higher microshear bond strength than the 7% TiO 2 -nt group (p = 0.034). Cohesive in material was the most representative failure mode for all groups.
Significance
The incorporation of TiO 2 -nt did not affect GIC’s adhesiveness to dentin, but improved its compressive strength at 5%. Furthermore, TiO 2 -nt decreased the percentage of weight loss after GIC’s surface wear.
1
Introduction
Glass ionomer cements (GIC) were first introduced into the market at the end of the 70 s, and since then their composition has been modified to become more appropriate for different clinical situations [ ]. The powder of conventional GIC is mainly composed by silica (SiO), alumina (Al 2 0 3 ) and calcium fluoride (CaF 2 ), with an alkaline composition, while the liquid contains polycarboxilic acid as a co-polymer with itaconic acid, tricarboxylic, maleic or tartaric, which increases the strength of the set cement and decrease its viscosity [ , ]. When mixing the powder and the liquid, the set reaction begins to form a hydrated salt, which acts as a bonding matrix for the glass particles [ , ]. Among the reported GIC properties, adhesion to dental structure, linear expansion coefficient similar to that of the tooth, biocompatibility and anticariogenic action due to the release of fluoride are highlighted [ , , ]. On the other hand, GIC has been reported to feature limitations, including high initial syneresis and imbibition that can result in dimensional changes, decrease of surface wear resistance and the formation of cracks and gaps [ ]. Such limitations may contribute to restoration failure with the possibility of bacterial proliferation and secondary caries lesions and/or restoration fractures, especially restorations performed as atraumatic restorative treatment (ART) in areas of great masticatory effort and involving multiple surfaces [ , ].
In order to overcome the above-mentioned limitations, the incorporation of different nanoparticles to GIC has been proposed, including metallic powders, montmorillonite clay, cellulose, zinc, silica, ceramics, ytterbium fluoride and barium sulfate, hydroxyapatite and fluoroapatite, aluminosilicate glass, casein phosphopeptide-amorphous calcium phosphate, bioactive glass and titanium dioxide [ , , ]. In general, it has been found that not all modifications have resulted in the desirable strengthening of GICs. For instance, metallic oxides and salts such as SrO and BaSO 4 did not affect GICs’ mechanical properties as they lacked the ability to increase the number of polysalt bridges and cross-linking within the glass matrix [ ]. Moreover, although bioactive glass alone led to an increased bioactivity, it decreased the material’s strength [ , ].
Titanium stands out because it is an inorganic additive, chemically stable, non-toxic and may present antimicrobial effects [ ]. Although some progress has been made with respect to the benefits of adding TiO 2 nanostructures to conventional GICs [ , , , ], there is a lack of evidence on the specific impact of TiO 2 -nt on the physico-mechanical properties of GICs. Main limitation of previous studies included the fact that they used spherical-shaped nanostructures, which tended to form clusters rather than permeate the GIC’s matrix [ , , ]. Tubular materials, such as TiO 2 -nt, are hollow nanostructures that feature a high surface-to-volume ratio [ ], which may contribute to improved surface energy, as well as better particle distribution, improving GIC’s overall properties. In a previous study, it has been demonstrated that GICs added with TiO 2 -nt presented improved surface microhardness and fluoride release at concentrations of 3% and 5%, while EDS analysis detected the presence of TiO 2 -nt into the material at 5% and 7% [ ]. However, additional studies are needed to determine the effect of TiO 2 at nano-tubular shape on GIC’s adhesion properties to dentin, compressive and flexural strength; providing the basis to establish restoration longevity [ , , ], as well as for the use of GICs in areas of high masticatory loads.
Therefore, the aim of this present study was to analyze the impact of the incorporation of the TiO 2 -nt to the conventional GIC on its physico-mechanical properties. The hypothesis of the current in vitro study were that the incorporation of TiO 2 -nt to the conventional high viscosity GIC would affect its: (a) compressive strength; (b) flexural strength; (c) microshear bond strength to dentin; (d) failure mode to dentin; (e) surface roughness before and after brushing simulation; and (f) weight loss before and after brushing simulation.
2
Materials and methods
2.1
Grouping of specimens
The factor under study was the incorporation of different concentrations of TiO 2 -nt (3%, 5% and 7% by weight) into a high viscosity GIC (Ketac Molar EasyMix™- 3 M/ESPE, Maplewood, Minnesota, USA). Four different experimental groups were obtained: Ketac Molar (KM) = control; KM + 3% TiO 2 -nt; KM + 5% TiO 2 -nt and KM + 7% TiO 2 -nt. Evaluated parameters included: compressive strength (n = 10/group); flexural strength (n = 18/group); microshear bond strength to dentin and failure mode (n = 20/group); roughness surface and weight loss before and after brushing simulation (n = 8/group). This study was conducted after approval of the research ethics committee (CAAE #64,262,617.3.0000.5374).
2.2
Specimens preparation
TiO 2 -nt at three different concentrations (3%, 5% and 7% in weight) were added to the powder of the GIC [aluminum-calcium fluorosilicate-lanthanum glass, 5% polycarbonate acid; shade A3], and then mixed to the liquid [polycarboxilic acid and tartaric acid] (Batch # 635,287; 638,396). Nanotubes (size ∼20 nm and diameter ∼10 nm), were formed by a single TiO 2 leaf spirally rolled and synthesized by the alkaline method [ ]. A precision scale accurate to 0.0001 g (Adventurer Oshaus, Parsippany, NJ, USA) was used to determine KM’s powder and TiO 2 -nt weights. After weighing the materials, nanotubes were manually added to the GIC powder at different concentrations (3%, 5%, 7% by weight) as previously proposed [ ]. Material agglutination was performed following the manufacturer’s instructions for powder/liquid ratio (1:1) using a metallic spatula and a block of waterproof paper. Specimens were prepared at room temperature (23 ± 1 °C and 50 ± 5% relative humidity in accordance with ISO 7489) following the recommendation by the manufacturer. In brief, GIC with or without TiO 2 -nt was placed in bipartite molds, inserted in a single increment with help from a Centrix syringe (Centrix Inc., Shelton, Connecticut, USA), and pressed between polyester strips (Proben, Catanduva, SP, Brazil) under a glass slide with a static load of approximately 200 g for 6 min. Next, specimens were covered with a thin layer of petroleum jelly (Rioquímica, São José do Rio Preto, SP, Brazil, batch#1,702,146) and stored for 24 h at 37 °C in relative humidity. After 24 h, the excess of petroleum jelly was gently removed with a soft paper from the specimen before conducting the tests [ ].
2.3
Compressive strength (CS)
Cylindrical specimens (6 × 4 mm) were prepared (n = 10/group, ISO 9917-1) and analyzed as previously described (ISO 9917-1) [ ]. CS was calculated (MPa) using the equation <SPAN role=presentation tabIndex=0 id=MathJax-Element-1-Frame class=MathJax style="POSITION: relative" data-mathml='CS=4PfπD2′>CS=4PfπD2CS=4PfπD2
C S = 4 P f π D 2
, where Pf represented the fracture load (Newtons), and D represented the diameter of the specimen (mm).
2.4
Flexural strength (FS)
Specimens (25 × 2 × 2 mm) were prepared (n = 18/group) and the FS test was performed based on the ISO 9917-2 [ ], subjected to three bending points on a 20 mm device in a universal testing machine. FS was determined (MPa) using the formula <SPAN role=presentation tabIndex=0 id=MathJax-Element-2-Frame class=MathJax style="POSITION: relative" data-mathml='σ=3Pl2bd2′>σ=3Pl2bd2σ=3Pl2bd2
σ = 3Pl 2b d 2
, where P (Newtons) represented the breaking load, L the distance between the two supports of 10 mm, b the sample width (mm) and d represented thickness (mm).
2.5
Microshear bond strength to dentin substrate (MSBS)
Human third molars (n = 20/group), extracted for orthodontic reasons and free from apparent caries, macroscopic cracks, abrasions and staining on the occlusal surface were selected (assessed by visual examination of radiographs with the assistance of magnifying lenses). Teeth were cleaned and frozen until processing time. Their roots were sectioned off 1 mm beyond the cement-enamel junction using a double-faced diamond saw (KG Sörensen, São Paulo, SP, Brazil). Crowns were longitudinally sectioned by a water-cooled diamond blade (Isomet 1000, Buehler Ltda., Lake Bluff, IL, USA), and each half was included in rigid PVC rings (Tigre, Joinville, SC, Brazil) with self-curing acrylic resin (JET-Classic, São Paulo, SP, Brazil). The inner surfaces were planned with 340 grit sandpaper (Buehler, Lake Bluff, IL, USA), polished with 600 grit sandpaper (Buehler) (ISO 6344-1:2014) [ ], rinsed with water spray, gently dried, and randomly assigned to one of the experimental groups. Next, dentin was actively conditioned with polyacrylic acid for 10 s, air-dried, and three siliconized polyvinyl cylinders (2 × 0.8 mm) were filled with the control and experimental materials on the medium dentin surface. MSBS values were obtained following the formula <SPAN role=presentation tabIndex=0 id=MathJax-Element-3-Frame class=MathJax style="POSITION: relative" data-mathml='τ=FA’>τ=??τ=FA
τ = F A
, where F represented the force obtained during the test (Newtons), and A represented the area subjected to the micro-shear test (mm²). The average value of the three cylinders for each group was used for statistical analysis [ , ].
2.6
Failure mode analyses
Failure mode produced after the MSBS tests was defined under a stereoscope (40× magnification, Eikonal EK3ST, São Paulo, SP, Brazil) and validated by SEM analysis (100× magnification, 15 kV, WD = 16 mm, spotsize = 25) (n = 20/group) as cohesive in dentin, cohesive in material or mixed. Failure modes were determined twice in a one-week interval by a calibrated examiner (Spearman correlation test with 95% intra-examiner coincidence).
2.7
Surface roughness and weight loss
Specimens were prepared (n = 8/group), polished with medium, fine and ultrafine aluminum oxide polishing discs (15 s/disc) (Sof-Lex Pop On, 3 M Dental Products, MN, USA), cleaned by ultrasound for 10 min (T1440D, Odontobrás, Ribeirão Preto, SP, Brazil), and submitted to brushing abrasion in an abrasion-testing machine (Biopdi, São Carlos, SP, Brazil) as previously reported [ , , ]. Briefly, thirty thousand cycles were performed at the speed of 250 strokes/min (complete forward and reverse movements), with a load of 200 g, with soft bristles tips (Palmolive, São Bernardo do Campo, SP, Brazil), corresponding to three years of brushing. Baseline and final data were collected in triplicate prior and after tooth brushing abrasion simulation. In the current study, CS, FS, MSBS tests were performed in a universal testing machine at a crosshead speed of 1 mm/min, and a load of 50 N (EMIC DL200, Campinas, SP, Brazil).
2.8
Surface characterization by scanning electron microscopy (SEM)
In order to determine the impact of TiO 2 -nt on the GIC’s structure, samples were submitted to SEM analysis (JEOL- JSM 5600 L V, Tokyo, Japan) (n = 3/group). Briefly, GIC samples were prepared as described above, with or without TiO 2 -nt, left to dry for 24 h, sputtered with a thin gold layer (Sputter Coater S150A, Japan), and analyzed at 1000× magnification in a working distance of 10 mm at 15 kV [ , ].
2.9
Statistical analysis
Data were analyzed using the Shapiro-Wilk normality test and Levene’s homoscedasticity (p ≤ 0.05). Analysis of variance (ANOVA) followed by the Tukey test was used to compare more than two groups, whereas the Dunnett’s test was used to access statistical differences between the control group (without TiO 2 -nt treatment) and TiO 2 -nt groups at different concentrations. The relative frequency (in %) followed by the chi-square test was used for failure mode. Analyzes were performed using a software (SPSS 21, Chicago, IL, USA), and the level of significance was 5% (α ≤ 0.05).
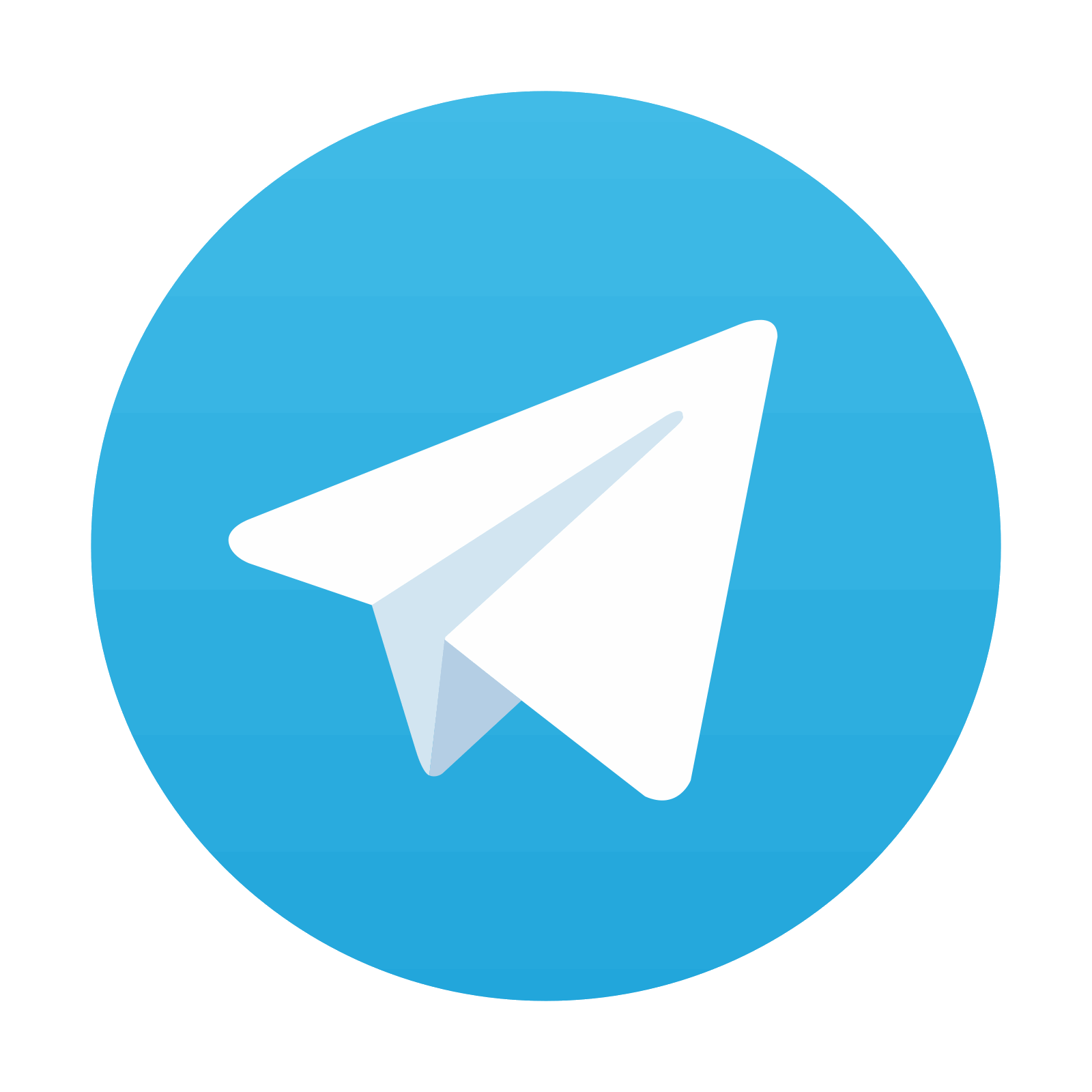
Stay updated, free dental videos. Join our Telegram channel

VIDEdental - Online dental courses
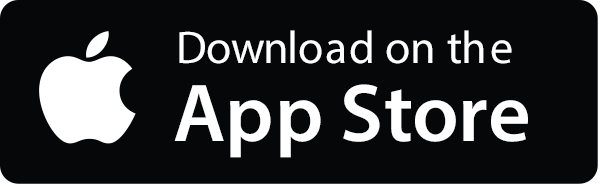
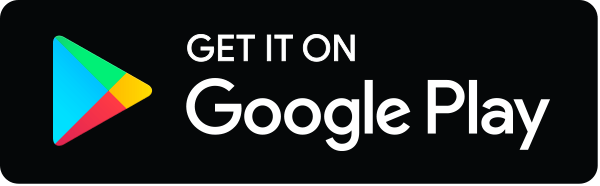