Mesenchymal stem cells (MSCs) are adult stem cells whose self-renewal, multipotency, and immunosuppressive functions have been investigated for therapeutic applications. MSCs have used for various systemic organ regenerative therapies, allowing rescue of tissue function in damaged or failing organs. This article reviews the regenerative and immunomodulatory functions of MSCs and their applications in dental, orofacial, and systemic tissue regeneration and treatment of inflammatory disorders. It also addresses challenges to MSC-mediated therapeutics arising from tissue and MSC aging and host immune response against allogenic MSC transplantation, and discusses alternative sources of MSCs aimed at overcoming these limitations.
- •
Mesenchymal stem cells (MSCs) are adult stem cells whose self-renewal, multipotency, and immunosuppressive functions have been investigated for therapeutic applications.
- •
Although initially isolated from systemic tissue sources, MSCs have also been isolated from dental and orofacial tissues yielding odontoblastic and cementoblastic differentiation capacities. These unique features facilitate their application for pulpal, periradicular, and mineralized dental tissue regeneration.
- •
MSCs have used for various systemic organ regenerative therapies, allowing rescue of tissue function in damaged or failing organs. MSCs also possess immunosuppressive functions that allow improved management and treatment of chronic inflammatory disorders. However, their propensity to undergo cellular senescence, as well as host immune response-mediated loss of potency of allogenicically transplanted MSCs, may limit their use in clinical settings.
Introduction
Interest in mesenchymal stem cells (MSCs) for tissue regenerative and other medical applications has stemmed from their multilineage and self-renewal capacity and ease of isolation. Since their initial discovery in the early 1960s, MSCs have been extensively characterized, and their tissue origin, morphology, self-renewal capacity, and multipotency have been well documented. Recently, new types of MSCs originating from dental and oral tissues have been identified and characterized. Although similar in morphology and replicative capacity, these dental MSCs (dMSCs) have been shown to possess distinct tissue and cell type-specific lineage paths, as well as immunosuppressive capacities, which have sparked interest in their application in dental and systemic tissue regeneration.
The first characterized MSCs were identified in bone marrow tissues. Although bone marrow stem cells (BMMSCs) are among the most widely characterized, MSCs have been derived from many tissues, including umbilical cord, adipose tissue, and hair follicle. Within the last decade and a half, a new type of MSC derived from dental and orofacial tissues has been identified. Among these MSC are dental pulp stem cells (DPSCs), stem cells from apical papilla (SCAPs), and periodontal ligament stem cells (PDLSCs), as well as stem cells from exfoliated deciduous teeth (SHEDs), and the more recently discovered dental follicle progenitor cells (DFPC). In contrast to BMMSCs, dMSCs primarily follow odontogenic (DPSC, SCAP, and SHED) or cementoblastic (PDLSC) rather than osteogenic development and are responsible for developing and repairing mineralized dental tissues during development and in response to disease. In addition, the authors’ laboratory recently reported generation of induced MSCs (iMSCs) from epithelial cells through retroviral transduction of gene ΔNp63α through process called epithelial–mesenchymal transition (EMT). These cells have demonstrated odontogenic and adipocytic differentiation properties under appropriate induction conditions. Since iMSCs can be derived from widely available tissues (eg, skin or mucosal keratinocytes), these cells may represent alternative source of MSCs, and further research will elucidate their therapeutic potential in oral and systemic conditions.
Morphologically, MSCs possess a spindle shape similar to that of fibroblasts. Upon serial subculture in vitro, dMSCs have been shown to develop morphologic changes consistent with cellular senescence, losing their spindle shape and exhibiting flattened, enlarged morphology. Although they exhibit a universal morphology, MSCs originate from different tissue sources, and not all cells collected from these tissues are MSCs. Several distinct cell surface markers have been identified for each class of MSCs that have been used to isolate MSCs from non-MSCs during tissue primary culture. CD44, CD73, and CD105 are expressed consistently across all BMMSCs and dMSCs, and selection of STRO-1 by flow cytometry has become the gold standard of MSC selection and isolation.
In addition to cell surface immunotype markers used to selectively isolate MSCs, several lineage-specific markers have also been identified. The osteogenic/odontoblastic lineage is among the most well characterized found in MSCs, and BMMSCs and dMSCs have been found to express common osteogenic/odontoblastic markers, such as collagen type 1, alkaline phosphatase, and bone sialoprotein. Altered gene expression of these markers has been used to investigate adverse effects of senescence in MSCs.
Unlike embryonic stem cells (ESCs), MSCs are adult stem cells possessing a committed lineage and lacking an indefinite self-renewal and totipotent capacity. MSCs have been shown to possess limited lifespan and ultimately undergo replicative senescence or aging in vitro. Self-renewal has been documented as ranging from 30 to 50 population doublings (PDs) in BMMSCs to more than 120 PDs in DPSC. Although self-renewal capacity of MSCs is more extensive than many somatic cells, studies have shown that, upon replicative senescence, dMSCs undergo morphologic and functional changes, in turn losing their multipotency and differentiative capacity. The limited self-renewal capacity and senescence-associated loss of function in MSCs subcultured in vitro may present an obstacle for therapeutic application.
Although MSCs may possess comparable phenotype and replicative capacities, their multipotency and differentiation capacity differ widely and are tissue specific. BMMSCs primarily possess osteogenic differentiation capacity and express osteogenic markers, whereas DPSC and SCAP follow odontoblastic lineages, and PDLSCs possess cementoblastic differentiation.
The committed lineage difference between these MSC is believed to be tissue directed. When transplanted, DPSC and SCAP form dentin–pulp-like complexes. For DPSC, this odontoblastic commitment facilitates the deposition of reparative dentin near pulp chamber in response to trauma or bacterial insult. In SCAP, this odontoblastic commitment facilitates tooth root formation during development. PDLSCs do not form dentin–pulp complexes and instead possess osteoblastic and cementoblastic lineages to regenerate periodontal tissue and maintain PDL integrity. Thus, the distinct lineages adopted by MSCs are believed to be tissue-specific to address the needs of the tissue of origin. Other than osteoblastic, odontoblastic, and cementoblastic lineages, MSCs have also been induced toward adipogenic, chrondogenic, and neurogenic lineages in vitro ( Tables 1 and 2 ). Alternate lineage commitment has been achieved in MSCs cultured in lineage-specific medium in vitro and evaluated by lineage-specific assays.
MSCs | Tissue Origin | Stem Cell Gene/Markers | Differentiation Markers | Multipotent Lineages |
---|---|---|---|---|
BMMSC | Bone marrow | STRO-1, Oct4, Nanog | Col I, Col III, ALP, BSP, OCN, OSX |
Osteogenic Adipogenic Neurogenic Chrondogenic |
DPSC | Dental pulp | STRO-1, Oct 4, TGFβR Endostatin, bFGF, FGFR3 |
Col I, Col III, ALP, BSP, DSP, OSX, Runx2 |
Odontoblastic Osteogenic Adipogenic Neurogenic Chondrogenic |
SCAP | Immature, developing apical papilla | STRO-1, Survivin, TGFβR, GFGR3, Endostatin, bFGF |
ALP, BSP, DSP, Runx2 | Odontoblastic Osteogenic Adipogenic Neurogenic |
PDLSC | Periodontal ligament | STRO-1, MUC18, TGFβR | ALP, BSP, OCN | Cementogenic Osteogenic Neurogenic Chondrogenic |
iMSC | Skin (keratinocytes) | Nanog, Lin28 | ALP, BSP, DSP, DMP-1, OCN, ON |
Osteogenic Adipogenic |
Lineage | Induction Condition | Phenotypic Marker | References |
---|---|---|---|
Osteogenic/odontoblastic | α-MEM, 10% FBS, ascorbic acid, β-GP, dexamethasone | Alizarin red staining Von Kossa staining |
|
Adipogenic | α-MEM, 20% FBS, dexamethasone | Oil Red – O staining | |
Neurogenic | DMEM, 20% FBS, FGF-2, BHA, forskolin, valproic acid, KCl, K252a, N2 supplement | Immunostaining (NSE, NeuN, Tau) | |
Chondrogenic | DMEM, insulin, transferin, selenious acid, BSA, linoleic acid, ascorbic acid | Alcian blue staining Safranin-O Staining |
Despite their limited replicative potential, MSCs continue to be investigated due to their immunomodulatory and immunosuppressive properties. MSCs have been shown to abrogate B cell antibody production, Th2 cell activation, and inflammatory cytokine production. Studies by Pevsner-Fischer and colleagues and Tomchuck and colleagues have shown Toll-like receptors 3 and 4 (TLR3, TLR4) to serve a critical role in MSC immunomodulation by regulation MSC inhibition of T lymphocytes. MSCs’ immunosuppressive capacity has also been shown to be regulated by proinflammatory cytokines (interferon [IFN]-γ, tumor necrosis factor [TNF]α, interleukin [IL]-1α, and IL-1β) that result in recruitment of MSCs and T cell mobilization. Spaggiari and colleagues demonstrated that MSCs possess the capacity to inhibit IL-2 mediated natural killer cell proliferation and cytokine production. MSCs have also been shown to lack functional MHC 2, which may further explain their immunosuppressive capacity. dMSC-specific immunosuppression has been demonstrated but not fully characterized.
Although complex, multifactorial, and highly regulated, MSCs’ immunosuppressive capacity has been of great interest due to therapeutic potential. Immunosuppression exhibited by MSCs may be manipulated to allow allogeneic tissue regeneration and host rejection-free transplantation. It may also facilitate treatment of degenerative conditions such as graft versus host disease and autoimmune diseases. This article will discuss the regenerative and immunomodulatory properties of MSCs and their applications in oral and systemic tissue regeneration and treatment of inflammatory disorders. It will also discuss challenges to MSC-mediated therapeutics that arise from aging of MSC donors and immunogenicity of allogeneic MSCs, and alternative sources of MSC aimed at overcoming these limitations.
Tissue engineering of dental structures and periodontium using dMSCs
Relevance of dMSCs for dentistry lies in their potential use in tissue regeneration of dental and dentofacial structures. The authors present 4 distinct categories of tissue regeneration for dental and periodontal structures, the underlying rationale, and current status of clinical translation ( Fig. 1 ). The first and simplest means of dental tissue regeneration is reparative dentine formation in dental pulp following direct pulp capping (DPC). Second, pulp revitalization allows for disinfection of necrotic, immature root canals and restoration of pulp-like tissues and pulp vitality. Third, pulp tissue engineering is aimed at regeneration of the pulp–dentin complex using exogenous dMSCs, biocompatible scaffolds, and growth factors. This approach might be considered an alternative to conventional root canal therapy (RCT) for mature permanent teeth with necrotic pulp and closed root apex. Finally, investigations are underway for tissue engineering of PDL for avulsed or extracted natural teeth and dental implants. The first 2 categories are using endogenous dMSCs, namely DPSC and SCAP, respectively, while the latter 2 categories rely on exogenous dMSCs for tissue engineering.
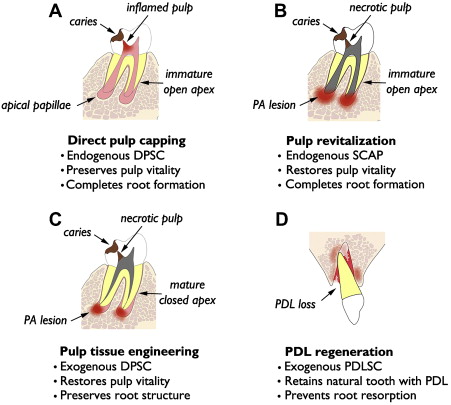
Direct Pulp Capping
Concept and practice of DPC goes back more than half century after the original publication by Castagnola and Orlay. Since then, DPC has been regarded as a viable alternative to RCT to preserve vital pulp following pulp exposure to caries or trauma. Examination of 359 DPC cases with follow-up ranging from 1 to 8 years demonstrated overall success of 61% when Ca(OH) 2 was used as the capping material. Several studies have demonstrated higher success with mineral trioxide aggregate (MTA) over those capped with Ca(OH) 2 . Mente and colleagues have shown 78% success with MTA pulp capping and 60% success with Ca(OH) 2 , while higher success rates (93%–98%) have been noted in other studies. Many of these studies demonstrate that long-term success of DPC depends, in part, on tooth type, capping material, incidence related to pulp exposure (eg, trauma vs caries), and patient’s age. For instance, Auschill and colleagues noted that the most successful DPC was seen with central incisors and in young (age 10–19 years) patients.
In many cases, DPC induces regenerative dentin formation, presumably from the resident DPSCs that were recruited to the site of defect. Earlier studies by Cvek demonstrated formation of hard dentin structure in permanent teeth with complex crown fracture. This report indicated that initial hard tissue formation was noted in 3 to 12 weeks, and complete bridge formation was noted in 3 to 6 months following partial pulpotomy. Likewise, in a routine DPC case, MTA placement led to notable formation of regenerative dentin in 6 months time in a young (9 years) patient ( Fig. 2 ). As mentioned earlier, DPC with Ca(OH) 2 is generally less successful than DPC capped with MTA. This may be attributed to the structural defects, known as tunnel defects, in hard tissue formed under Ca(OH) 2 , causing bacterial leakage. As such, dental pulp retains robust healing capacity through differentiation of DPSCs into odontoblasts, and DPC has been shown to be highly successful with newer materials like MTA. Clinically, DPC should be considered as a viable alternative to RCT, especially for immature teeth with open apices in young children.
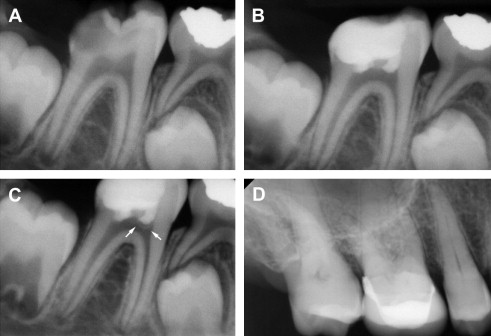
Pulp Revitalization
Recent studies demonstrate the feasibility of revitalizing chronically abscessed necrotic teeth through regenerative endodontics procedures. This approach would be greatly beneficial for immature teeth with divergent apices, because revitalization of such teeth would allow for continued root development and apical closure. On the contrary, conventional treatment would involve apexification, which permanently arrests root development, leaving the tooth more susceptible to fracture. A study by Bose and colleagues demonstrated significantly increased root length and thickness in teeth after revitalization when compared with those that had received apexification. Thus, there is clear justification for this new approach for immature, necrotic teeth.
While varied clinical protocols are reported, the unifying theme of pulp revitalization procedures is to disinfect the root canals using either Ca(OH) 2 or triple antibiotic paste, induce intracanal bleeding through the apical foramen, and allow endogenous MSCs to resume odontogenic differentiation and complete the root formation. Underlying assumptions are that there is residual pool of SCAPs surviving the chronic infection and that these cells are reactivated once the source of inflammation is resolved. A recent study showed enriched population of CD73+ and CD105+ MSCs in intracanal blood during revitalization procedure. Thus, evidence suggests recruitment of MSCs during revitalization into the root canal, although the source of these cells and their role in pulp–dentin reconstitution remain completely unknown.
Validity of pulp revitalization as routine endodontic therapy requires understanding of long-term outcome and predictability. So far, this information is generally limited to several case reports, with follow-up periods ranging from a few months to 2 to 3 years postoperatively. However, preliminary results shown in the case reports are striking. Chueh and colleagues report successful resolution of apical lesions and root development in 23 necrotic immature permanent teeth after the revitalization procedure using Ca(OH) 2 . In another study, revitalization was performed using platelet-rich plasma (PRP) on a necrotic maxillary second premolar that had regained sensitivity to cold and electric pulp testing. Clearly, growing evidence is in favor of revitalization as a viable alternative to apexification, although systematic evaluation of treatment outcomes with larger numbers of cases and longer follow-up periods is needed.
Pulp Tissue Engineering
Both DPC and revitalization discussed previously harness the regeneration capacities of endogenous dMSCs, either representing DPSCs or SCAPs, respectively. Revitalization of necrotic mature teeth with closed apex may necessitate pulp tissue engineering, which may require biodegradable scaffolds, addition of exogenous dMSCs, and growth factors (eg, TGF-β, BMP-7, and bFGF). Pulp tissue engineering is far more complicated than revitalization using endogenous dMSCs and is being developed as an alternative to conventional RCT. Although literature supports high success rates upwards of 90%, structural defects are the leading cause of tooth extraction among endodontically treated teeth. Vire reported that 59.4% of endodontically treated teeth were extracted due to prosthetic failure (eg, crown fracture, root fracture, and recurrent decay), whereas 8.6% were extracted due to endodontic failure. Such structural failure may simply be due to removal of dentinal matter during cleaning and shaping, rather than any substantive changes to dentin by lack of vitality. Sedgley and Messer showed no significant difference in dentinal shear strength, toughness, or fracture resistance between vital dentin and those treated endodontically. Thus, structural imperfections associated with conventional RCT may be avoided by devising regenerative therapies, which may reinforce dentinal structure.
Recent studies demonstrated proof-of-concept for pulp tissue engineering using extracted root model transplanted onto immunocompromised animals. Huang and colleagues used a hollowed root filled with polylactide/glicolide (PLG) scaffolds and DPSCs, and showed pulp regeneration and dentin formation inside the root canal wall. Subsequently, the tooth slice model developed by Sakai and colleagues showed de novo dentinogenesis in artificial pulp regenerated with poly-L-lactic acid (PLLA) and DPSCs. Pulp regeneration therefore allowed for new hard tissue formation in dental pulp that may aid in reinforcing structural stability. Other advantages of pulp tissue engineering may include reinstating immune defense mechanisms and innervation for environmental stimuli, including temperature changes, excessive load, and bacterial invasion. These functions of dental pulp are critical for protecting the tooth structure from potential fractures and recurrent decay. Conventional RCT offers no functional restoration of pulp, and such deficiencies may account for the need to extract endodontically treated teeth. At present, there is no clinical study demonstrating successful restoration of functional pulp through tissue engineering, although preclinical studies using dMSC and the scaffolds have yielded promising results.
PDL Regeneration
PDLSCs have been isolated from extracted human teeth and have shown ability to form cementum- and PDL-like structures when transplanted in vivo. These cells opened the possibility of PDL regeneration on root surfaces of extracted or avulsed teeth. Traditionally, tooth avulsion and replantation have had poor long-term survival resulting from root resorption and ankylosis. Donaldson and Kinirons showed that 68% of all replanted teeth undergo root resorption and that resorption is enhanced by extended dry-time (ie, duration of tooth being out of socket) and presence of contamination. PDL regeneration may prevent root resorption in avulsion–replantation cases or intentional tooth replantation or transplantation. Like pulp tissue engineering, PDL regeneration would require an exogenous source of dMSCs. A preclinical animal study was recently published by Dangaria and colleagues to demonstrate feasibility of PDL regeneration. This study used the extraction–replantation model using rat maxillary molars with or without PDLSC coating on the root surface; when evaluated 6 months after replantation, almost complete resorption occurred on the denuded root surface. However, those teeth with PDLSC coating revealed newly formed PDL structure and absence of root resorption. Thus, PDLSCs are able to regenerate PDL tissue on a replanted tooth. Further development of this technology will allow better management of avulsion–replantation cases and possibly extraoral manipulation of teeth for routine dental work. In addition, PDL regeneration is a possibility on titanium implant surfaces, as recently demonstrated in an animal model. One clinical study demonstrated cell-coated dental implant placement in the mandible and PDL formation around the implant surface. This is the proof-of-concept study for ligament-anchored implants or ligaplant for clinical use in people. Among many, 1 obvious benefit for ligaplant would be the possibility to mobilize dental implants using orthodontic appliances.
Tissue engineering of dental structures and periodontium using dMSCs
Relevance of dMSCs for dentistry lies in their potential use in tissue regeneration of dental and dentofacial structures. The authors present 4 distinct categories of tissue regeneration for dental and periodontal structures, the underlying rationale, and current status of clinical translation ( Fig. 1 ). The first and simplest means of dental tissue regeneration is reparative dentine formation in dental pulp following direct pulp capping (DPC). Second, pulp revitalization allows for disinfection of necrotic, immature root canals and restoration of pulp-like tissues and pulp vitality. Third, pulp tissue engineering is aimed at regeneration of the pulp–dentin complex using exogenous dMSCs, biocompatible scaffolds, and growth factors. This approach might be considered an alternative to conventional root canal therapy (RCT) for mature permanent teeth with necrotic pulp and closed root apex. Finally, investigations are underway for tissue engineering of PDL for avulsed or extracted natural teeth and dental implants. The first 2 categories are using endogenous dMSCs, namely DPSC and SCAP, respectively, while the latter 2 categories rely on exogenous dMSCs for tissue engineering.
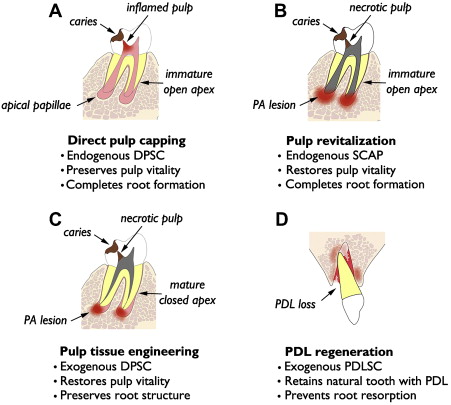
Direct Pulp Capping
Concept and practice of DPC goes back more than half century after the original publication by Castagnola and Orlay. Since then, DPC has been regarded as a viable alternative to RCT to preserve vital pulp following pulp exposure to caries or trauma. Examination of 359 DPC cases with follow-up ranging from 1 to 8 years demonstrated overall success of 61% when Ca(OH) 2 was used as the capping material. Several studies have demonstrated higher success with mineral trioxide aggregate (MTA) over those capped with Ca(OH) 2 . Mente and colleagues have shown 78% success with MTA pulp capping and 60% success with Ca(OH) 2 , while higher success rates (93%–98%) have been noted in other studies. Many of these studies demonstrate that long-term success of DPC depends, in part, on tooth type, capping material, incidence related to pulp exposure (eg, trauma vs caries), and patient’s age. For instance, Auschill and colleagues noted that the most successful DPC was seen with central incisors and in young (age 10–19 years) patients.
In many cases, DPC induces regenerative dentin formation, presumably from the resident DPSCs that were recruited to the site of defect. Earlier studies by Cvek demonstrated formation of hard dentin structure in permanent teeth with complex crown fracture. This report indicated that initial hard tissue formation was noted in 3 to 12 weeks, and complete bridge formation was noted in 3 to 6 months following partial pulpotomy. Likewise, in a routine DPC case, MTA placement led to notable formation of regenerative dentin in 6 months time in a young (9 years) patient ( Fig. 2 ). As mentioned earlier, DPC with Ca(OH) 2 is generally less successful than DPC capped with MTA. This may be attributed to the structural defects, known as tunnel defects, in hard tissue formed under Ca(OH) 2 , causing bacterial leakage. As such, dental pulp retains robust healing capacity through differentiation of DPSCs into odontoblasts, and DPC has been shown to be highly successful with newer materials like MTA. Clinically, DPC should be considered as a viable alternative to RCT, especially for immature teeth with open apices in young children.
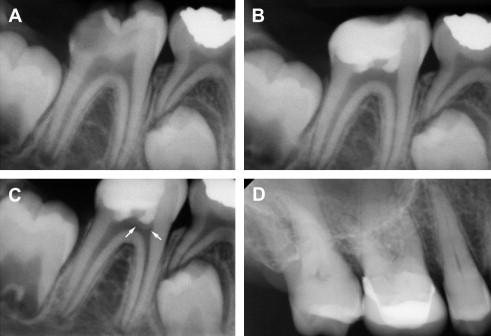
Pulp Revitalization
Recent studies demonstrate the feasibility of revitalizing chronically abscessed necrotic teeth through regenerative endodontics procedures. This approach would be greatly beneficial for immature teeth with divergent apices, because revitalization of such teeth would allow for continued root development and apical closure. On the contrary, conventional treatment would involve apexification, which permanently arrests root development, leaving the tooth more susceptible to fracture. A study by Bose and colleagues demonstrated significantly increased root length and thickness in teeth after revitalization when compared with those that had received apexification. Thus, there is clear justification for this new approach for immature, necrotic teeth.
While varied clinical protocols are reported, the unifying theme of pulp revitalization procedures is to disinfect the root canals using either Ca(OH) 2 or triple antibiotic paste, induce intracanal bleeding through the apical foramen, and allow endogenous MSCs to resume odontogenic differentiation and complete the root formation. Underlying assumptions are that there is residual pool of SCAPs surviving the chronic infection and that these cells are reactivated once the source of inflammation is resolved. A recent study showed enriched population of CD73+ and CD105+ MSCs in intracanal blood during revitalization procedure. Thus, evidence suggests recruitment of MSCs during revitalization into the root canal, although the source of these cells and their role in pulp–dentin reconstitution remain completely unknown.
Validity of pulp revitalization as routine endodontic therapy requires understanding of long-term outcome and predictability. So far, this information is generally limited to several case reports, with follow-up periods ranging from a few months to 2 to 3 years postoperatively. However, preliminary results shown in the case reports are striking. Chueh and colleagues report successful resolution of apical lesions and root development in 23 necrotic immature permanent teeth after the revitalization procedure using Ca(OH) 2 . In another study, revitalization was performed using platelet-rich plasma (PRP) on a necrotic maxillary second premolar that had regained sensitivity to cold and electric pulp testing. Clearly, growing evidence is in favor of revitalization as a viable alternative to apexification, although systematic evaluation of treatment outcomes with larger numbers of cases and longer follow-up periods is needed.
Pulp Tissue Engineering
Both DPC and revitalization discussed previously harness the regeneration capacities of endogenous dMSCs, either representing DPSCs or SCAPs, respectively. Revitalization of necrotic mature teeth with closed apex may necessitate pulp tissue engineering, which may require biodegradable scaffolds, addition of exogenous dMSCs, and growth factors (eg, TGF-β, BMP-7, and bFGF). Pulp tissue engineering is far more complicated than revitalization using endogenous dMSCs and is being developed as an alternative to conventional RCT. Although literature supports high success rates upwards of 90%, structural defects are the leading cause of tooth extraction among endodontically treated teeth. Vire reported that 59.4% of endodontically treated teeth were extracted due to prosthetic failure (eg, crown fracture, root fracture, and recurrent decay), whereas 8.6% were extracted due to endodontic failure. Such structural failure may simply be due to removal of dentinal matter during cleaning and shaping, rather than any substantive changes to dentin by lack of vitality. Sedgley and Messer showed no significant difference in dentinal shear strength, toughness, or fracture resistance between vital dentin and those treated endodontically. Thus, structural imperfections associated with conventional RCT may be avoided by devising regenerative therapies, which may reinforce dentinal structure.
Recent studies demonstrated proof-of-concept for pulp tissue engineering using extracted root model transplanted onto immunocompromised animals. Huang and colleagues used a hollowed root filled with polylactide/glicolide (PLG) scaffolds and DPSCs, and showed pulp regeneration and dentin formation inside the root canal wall. Subsequently, the tooth slice model developed by Sakai and colleagues showed de novo dentinogenesis in artificial pulp regenerated with poly-L-lactic acid (PLLA) and DPSCs. Pulp regeneration therefore allowed for new hard tissue formation in dental pulp that may aid in reinforcing structural stability. Other advantages of pulp tissue engineering may include reinstating immune defense mechanisms and innervation for environmental stimuli, including temperature changes, excessive load, and bacterial invasion. These functions of dental pulp are critical for protecting the tooth structure from potential fractures and recurrent decay. Conventional RCT offers no functional restoration of pulp, and such deficiencies may account for the need to extract endodontically treated teeth. At present, there is no clinical study demonstrating successful restoration of functional pulp through tissue engineering, although preclinical studies using dMSC and the scaffolds have yielded promising results.
PDL Regeneration
PDLSCs have been isolated from extracted human teeth and have shown ability to form cementum- and PDL-like structures when transplanted in vivo. These cells opened the possibility of PDL regeneration on root surfaces of extracted or avulsed teeth. Traditionally, tooth avulsion and replantation have had poor long-term survival resulting from root resorption and ankylosis. Donaldson and Kinirons showed that 68% of all replanted teeth undergo root resorption and that resorption is enhanced by extended dry-time (ie, duration of tooth being out of socket) and presence of contamination. PDL regeneration may prevent root resorption in avulsion–replantation cases or intentional tooth replantation or transplantation. Like pulp tissue engineering, PDL regeneration would require an exogenous source of dMSCs. A preclinical animal study was recently published by Dangaria and colleagues to demonstrate feasibility of PDL regeneration. This study used the extraction–replantation model using rat maxillary molars with or without PDLSC coating on the root surface; when evaluated 6 months after replantation, almost complete resorption occurred on the denuded root surface. However, those teeth with PDLSC coating revealed newly formed PDL structure and absence of root resorption. Thus, PDLSCs are able to regenerate PDL tissue on a replanted tooth. Further development of this technology will allow better management of avulsion–replantation cases and possibly extraoral manipulation of teeth for routine dental work. In addition, PDL regeneration is a possibility on titanium implant surfaces, as recently demonstrated in an animal model. One clinical study demonstrated cell-coated dental implant placement in the mandible and PDL formation around the implant surface. This is the proof-of-concept study for ligament-anchored implants or ligaplant for clinical use in people. Among many, 1 obvious benefit for ligaplant would be the possibility to mobilize dental implants using orthodontic appliances.
Regenerative therapies using MSCs for systemic diseases
Recent advances in the use of MSC-mediated therapies hold great promises to potentially managing and treating systemic diseases. Systemic applications of MSC therapies are grossly divided into 2 categories, regenerative and immunomodulatory therapies. Regenerative therapy refers to a therapeutic modality in which structures and functions of an organ are regenerated to the clinically acceptable level with MSCs. Immunomodulatory therapy uses immunomodulatory characteristics of MSCs capable of intervening both innate and adaptive immunity. This section will discuss about use of MSCs for both therapeutic purposes.
The main goals of using MSCs for regenerative therapies for systemic diseases include restoration of forms or functions of cells, tissues, and organs that are missing or malfunctioning. MSCs have been shown to differentiate into different types of cells in vitro ( Table 3 ). In addition, preclinical animal studies provided rationales to push the use of MSCs at the clinical trial levels to regenerate functionally failed organs. In this section, the authors will discuss potential role of MSCs for functional restoration of critical organ systems, such as skin, cardiac muscle, pancreas, and liver.
Organ/Cells | MSC Sources | Induction Conditions | Differentiation Markers | References |
---|---|---|---|---|
Skin/keratinocytes | Hematopoietic | Keratinocyte-conditioned medium | ↑KRT14 ↑TGM1 |
|
Heart/cardiomyocytes | Bone marrow | 5-azacytidine | ↑ action potential ↑ Nkx2.5/Csx, GATA4 ↑ MEF-2A, MEF-2D |
|
Bone marrow | Insulin, dexamethasone, ascorbic acid | ↑ Nkx2.5/Csx, GATA4 ↑ MEF-2A, MEF-2D |
||
Pancreas/β-cells | Bone marrow | 1. High glucose (for expansion) 2. Nicotinamide, exendin4, glucose (for differentiation) |
↑ insulin 1 and 2 ↑Glut2, PDX-1, Pax6 |
|
Bone marrow | Rat pancreatic extract (RPE) | ↑ insulin 1 and 2 ↑glucagon ↑pancreatic polypeptide ↑somatostatin |
||
Bone marrow | 1. Ectopic expression of IPF1, HLXB9, and FOXA2 2. Islet-conditioned medium |
↑FOXA2, PAX4, and ISL1 ↑ insulin 1 and 2 ↑glucagon |
||
Liver/hepatocytes | Bone marrow | 1. ITS, FGF, EGF (for expansion) 2. HGF, NTA, Dexamethazone (for differentiation) |
↑albumin, HepPar-1, α-fetoprotein, KRT18, KRT19, nestin | |
Bone marrow | 1. IGF-I, HGF, dexamethasone (for expansion) 2. Oncostatin M (for differentiation) |
↑albumin, α-fetoprotein |
Skin Wound Closure
Currently, the standard of care for skin defects is split-thickness autograft, but its practice is limited to availability and sizes of wounds. For large skin defects such as traumatic, burned, or nonhealing wounds, regenerative therapies are promising therapeutic modalities. The primary goals of skin regenerative therapies are to restore physical barriers to prevent infection and the loss of body fluid and to provide esthetically acceptable appearance. Skin regenerative therapies are largely divided into 3 categories: (1) artificially bioengineered skin substitutes, (2) cell-based therapies, and (3) combination of skin substitutes and cell-base therapies. Integra is a bilayer artificial skin substitute with silicone in the external layer and bovine collagen/chondroitin sulfate matrix in the inner layer. Integra serves as a temporary matrix, which allows for ingrowth of cells including fibroblasts, and it has been used and widely accepted as the prototypical biomaterials for restoration of skin defects. Cultured epithelial autografts (CEAs) are cell-based therapies in which keratinocytes and fibroblasts are integrated onto a collagen sheet to reconstitute skin-like constructs. CEA is advantageous, because of its autologous tissue constructs; however, its use is limited due to high cost, the fragility of the graft materials, and increased total costs including hospital care for the follow-ups. Studies have shown that combination of CEA and Integra allows for rapid healing and complete restoration of the skin at the wound site ; however, complete skin regeneration is not achievable because (1) cultured keratinocytes and fibroblasts contain minimal, if not all, amounts of multipotent stem cells required to reconstitute fully functional skin, and (2) stem cells are most likely needed to reconstitute different functional components of skin including hair follicles and sweat glands.
Recent studies demonstrate that skin-regenerative therapies using MSCs are potentially promising. Several groups reported complete closure of chronic wounds when freshly prepared or in vitro-cultured bone marrow-derived cells were topically applied to the wounded sites. Bone marrow MSC impregnated onto collagen matrix has been shown to be effective in closure of chronic wounds. MSC derived from adipose tissues were also used with or without fibrin glue to expedite closure of perianal fistula, and the long-term follow-ups revealed that 17 out of 24 patients treated with MSCs had the fistula remained closed, whereas 3 out of 25 patients treated without MSCs had the open fistula. Therefore, use of MSCs to enhance cutaneous wound healing in the clinical settings is a promising therapeutic modality in skin regeneration.
Despite the promising clinical outcomes of MSC-mediated therapy on chronic wound healing, direct conversion of MSCs to the skin epithelial cells (eg, keratinocytes) as a underlying mechanisms has only been recently suggested. Fujita and colleagues showed that hematopoietic-derived MSCs exhibited keratinocyte-specific markers when either co-cultured with keratinocytes or cultured in keratinocyte-conditioned medium. However, because expedited cutaneous wound healing mediated by MSCs is most likely associated with enhanced reepithelialization, cellularity, and angiogenesis, whether such results are associated with de novo conversion of MSCs into functional keratinocytes (eg, formation of epithelial barriers) and play an important role in wound healing of the skin in vivo requires further examination.
Cardiac Muscle
Progressive functional failure of heart muscle after a myocardial infarction is among the major heart problems in elderly population worldwide. Because the cardiac muscle has long been considered as a postmitotic organ without regenerative potential, the infarcted heart fails to regenerate contractile cardiac tissues but is replaced by noncontractile fibrous tissues. Therefore, the goal of cardiac muscle regeneration is to rejuvenate the cardiac muscles and to restore the contractile forces.
Recent in vitro studies suggest that MSCs can be induced to differentiate into contractile cardiomyocytes. Fukuda and Makino and colleagues showed that MSC treated with 5-aza-cyidine became cardiomyocytes as demonstrated by increased expression of cardiac-specific transcription factors such as GATA4 and Nkx2.5. MSCs cultured in medium containing insulin, dexamethasone, and ascorbic acid also differentiated into cardiomyocytes, suggesting that MSC may serve as an adequate source for cardiac muscle regeneration therapy.
Preclinical animal studies demonstrated that MSCs improve cardiac function after myocardial infarction, although the mechanisms behind such improvement are incompletely understood. Miyahara and colleagues showed that a sheet of MSCs transplanted onto the scarred myocardium after myocardial infarction in mice grew to form a thick stratum and improved cardiac function. MSCs directly injected into the infarcted cardiac muscle in mice also showed improved cardiac function.
As of 2011, there were total of 19 clinical trials for MSC-mediated heart therapy for heart diseases. Recent phase 1 clinical study showed significant improvement in cardiac contractility as determined by left ventricular ejection fraction. Nonetheless, there are still unsolved challenges such as transient enhancement of cardiac contractility without structural repair, lack of evidence in long-term survival, and efficient delivery of the MSCs to the donor sites.
Pancreatic β-Cells
The pancreas is an important organ that produces insulin, a critical factor that regulates the blood glucose levels. Insulin is produced by β-cells within the pancreatic islets of Langerhans, and destruction of β-cells leads to hypoglycemia and type 1 diabetes mellitus. Patients with type 1 diabetes mellitus are susceptible to vascular damages, and, over period of time, develop chronic complications such as retinopathy, neuropathy, nephropathy, and cardiovascular diseases.
Current treatment of type 1 diabetes mellitus is exogenous insulin administration. Whole-organ transplantation has been performed on those who are nonresponsive to exogenous insulin-based treatment ; however, it is limited by systemic infection and lifelong immunosuppression secondary to organ transplantation, and relapse due to incomplete achievement for insulin independence.
Because type 1 diabetes mellitus is primarily due to the functional loss of a single cell type, β-cells within the pancreatic islets of Langerhans, MSC-mediated therapy is a plausible treatment modality. In vitro, MSCs have been demonstrated to be reprogrammed to insulin-producing cells. Several groups demonstrated that MSCs derived from human or rodent bone marrow can be differentiated into insulin-producing cells. MSCs derived from different anatomic sources have also also been shown to be differentiated into insulin-producing cells. It additionally has been demonstrated that these insulin-producing cells are responsive to glucose stimulation, suggesting MSCs are the promising alternative cell population that can functionally replace damaged pancreatic β-cells.
Preclinical studies using animal models demonstrated that MSCs can functionally and structurally replace pancreatic β-cells in vivo. Ianus and colleagues genetically engineered MSCs that produce enhanced green fluorescent protein (EGFP) when the insulin gene is actively transcribed. These MSCs were transplanted onto the immune-suppressed mice, and EGFP-positive cells were detected in the pancreatic islets. When these cells were explanted and grown in vitro, they produced glucose-dependent and incretin-enhanced insulin secretion, suggesting MSCs can be differentiated into pancreatic β-cells to produce insulin in vivo. However, subsequent studies by other groups showed different results; transplanted MSCs became pancreatic β-cells, but no insulin was secreted from these cells. Nonetheless, MSC transplantation resulted in endogenous pancreatic tissue repair, suggesting that MSC-mediated therapy is a viable therapeutic modality for nonfunctional or damaged pancreas such as in type 1 diabetes mellitus.
Liver
The liver is a vital organ that detoxifies toxic substances. Damages to the liver by portal hypertension, chronic liver failure, or viral- or nonviral-mediated cancer lead to fibrosis. The end-stage fibrosis or cirrhosis requires liver transplantation. However, similar to pancreas transplantation, the limitations to liver transplantation are availability of the liver donors, immune rejection, surgical intervention, and high costs. Cell-based therapy can be beneficial as an alternative to transplantation.
Several groups reported successful differentiation of MSCs into the hepatocytes in vitro. Chivu and colleagues used a 2-stage method to differentiate MSCs to hepatocytes. Histone deacetylase, valproic acid, and cytokines such as hepatocyte growth factor (HGF) and insulin-like growth factor 1 (IGF-1) were also used for hepatogenic differentiation.
Preclinical animal studies also demonstrated the potential use of MSCs to manage liver fibrosis. In a D-galatosamine induced rat model of acute liver injury, MSC-mediated therapy caused a reduction of apoptotic hepatocellular death and enhancement of hepatocyte proliferation. The improvement of liver functions by MSCs has been demonstrated in other liver injury models. Similarly, recent clinical trials demonstrated efficacy and safety of MSC-mediated liver generation therapy, suggesting that MSC-mediated therapy for liver failures is promising therapeutic modality.
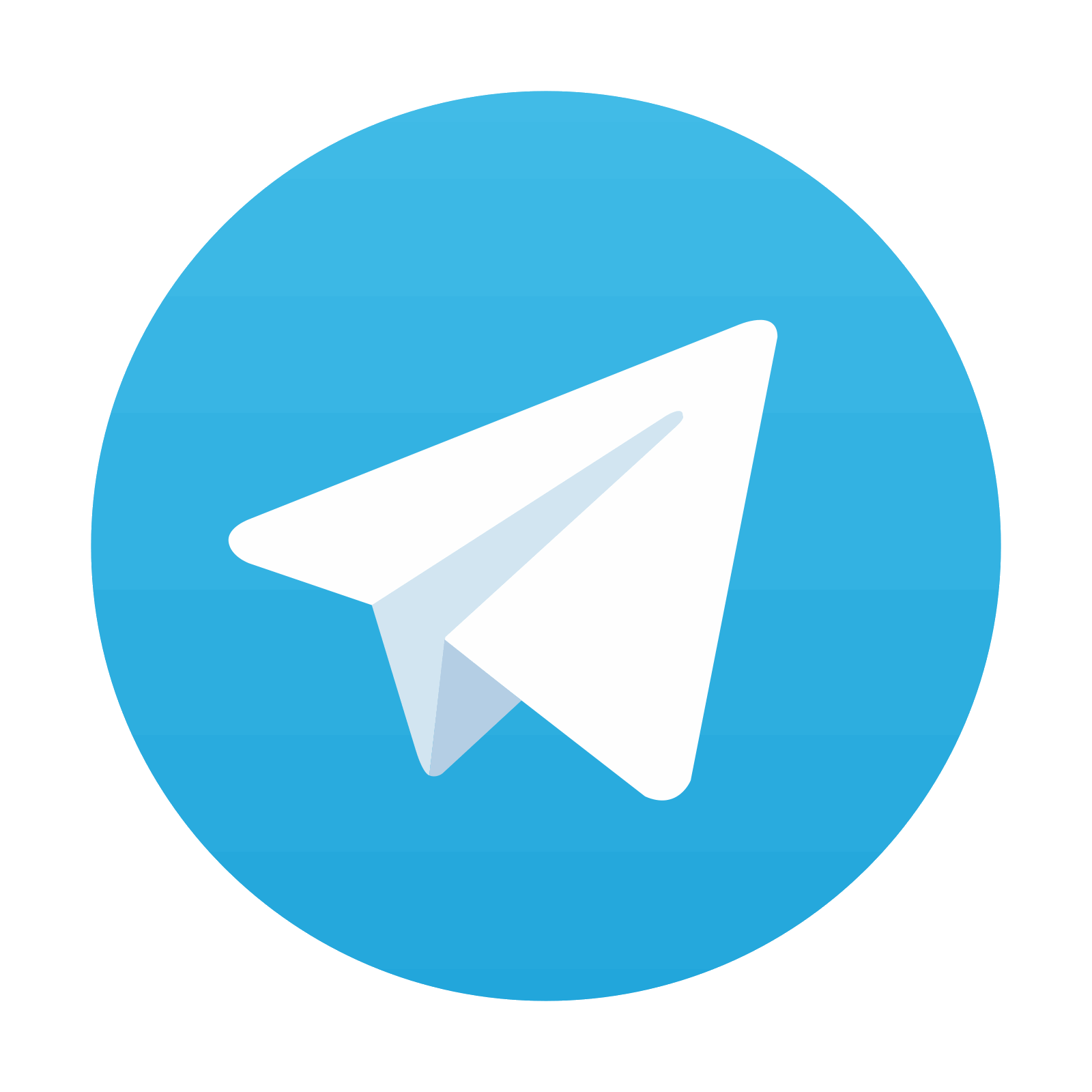
Stay updated, free dental videos. Join our Telegram channel

VIDEdental - Online dental courses
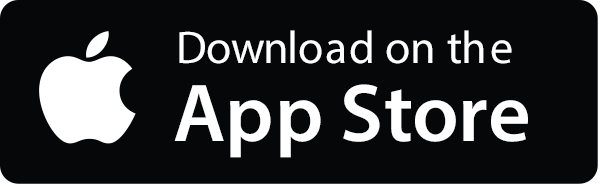
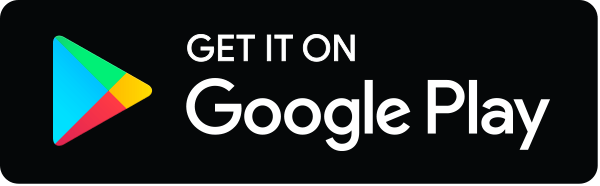