Fig. 8.1
Primary HSACs encapsulated in HA hydrogel. Single acinar cells are encapsulated and grown over 5 days to form multicellular spheroids indicated by (a) nuclear staining and delineated by (b) filamentous actin staining. (c) Confocal micrograph of merged image and (d) DIC image of the hydrogel
The use of progenitor cells poses as an attractive model for regenerating glandular tissue. Work by Knox et al., Nanduri et al., Kishi et al., and Lombaert et al. has shown much promise in this field [15, 89–92]. Transcription factors Ascl3 and Sox2, structural protein K5, and stem cell growth factor receptor c-kit have been used to identify progenitor and stem cell populations. Lombaert et al.’s report on the ability of a small population of c-kit-positive cells to rescue salivary gland morphology and function in irradiation glands has led the way to the possibility of successful stem cell therapy [90]. These studies can lead us to a combination approach where stem/progenitor marker-expressing cells are combined with just the correct bioactive scaffold that can be used to generate glands in vitro.
Efforts to Implement Vasculature and Innervation in a Tissue-Engineered Gland
One of the major challenges in tissue engineering is avoiding necrosis of implanted tissue. In order to ensure adequate nutrient flow to the engineered tissue, vascular supply is essential [93].
To overcome this problem, several strategies including overexpression of the angiogenic factor vascular endothelial growth factor (VEGF), incorporation of VEGF in engineered implants, or controlled release of VEGF from bioactive scaffolds have been sought [93–96]. Recent studies have suggested inclusion of mesenchymal stem cells to improve vascularization of tissues. Additionally, mesenchymal stem cells seeded in 3D environments form spheroid aggregates that have been found to secrete increased levels of VEGF and FGF-2 [93, 97].
Salivary fluid secretion is stimulated upon the binding of acetylcholine, a muscarinic agonist, which is released by parasympathetic nerves. Similarly, salivary protein secretion is dependent on the binding of β-adrenergic agonists, which are released by the sympathetic nerves [8, 13, 98]. Inclusion of the nerve growth factor (NGF), a neurotrophin, into implanted scaffolds has shown promise in nerve regeneration [99]. Complete functionality of salivary gland tissue cannot be achieved without adequate innervation of the engineered glands.
Modeling Salivary Gland Disorders
Studying disease-associated mechanisms is crucial for advancement toward generating new treatment, cures, and diagnostic tools for disease. Specifically, studying diseased tissues can yield new biomarkers for disease, or validate currently existing ones, and ultimately advance the establishment of accurate diagnostic tools. Salivary diagnostic tools would be a more feasible, economical, and accessible form of monitoring the patient’s health. Diagnostic exams could be performed in the comfort of one’s home due to how easy it is to collect saliva without the use of needles. However, in order to establish salivary diagnostic tools as the platform for monitoring health status, biomarkers must be adequately validated, and biomarker information must be easily accessible to all [100].
Biological models of diseased salivary gland tissue can be an available tool that can provide useful information on salivary gland diseases such as Sjögren’s syndrome, radiation-affected glands, salivary gland cancers, and the like. An in vitro model of Sjögren’s syndrome, an autoimmune disease in which your body’s immune system attacks the lacrimal and salivary glands, could be accomplished by isolating healthy salivary acinar cells, growing them in an in vitro system, and comparing salivary secretions from before and after coculturing with lymphocytes or treatment with an immune system product in question [101]. Such a model would be beneficial for studying the associated mechanisms because of the ability to control the added immune system products and more precisely measure an outcome. For example, this model could be used to further investigate the role of TGF-β (beta) in preventing autoimmune responses by suppressing proliferation and differentiation of lymphocytes [102]. Once an in vitro model is established, treatments could be tested in vitro before investing in in vivo animal models.
The effects of irradiation therapy on salivary glands can also be studied using an in vitro model. Approximately 40,000 head and neck cancer patients suffer from radiation-induced xerostomia every year [103]. Chemoradiation of head and neck cancers leads to a significant reduction in acinar cells, ductal metaplasia, and fibrosis [104]. The mechanism in which radiation therapy causes acinar cell loss needs to be further investigated. Furthermore, an in vitro salivary gland model could be used as a method to study radiation-induced effects on acinar cells and discover possible effective treatments.
Three-dimensional tissue culture systems also allow for the ex vivo growth of salivary gland tumor specimens and the study of the mechanisms associated with salivary gland cancers. The majority of tumors affecting the major salivary glands are benign and include pleomorphic adenomas and Warthin’s tumor. Malignant tumors are rare in salivary glands and mostly affect people over the age of 60; these include mucoepidermoid carcinoma, adenocarcinoma, squamous cell carcinoma, malignant mixed tumors, adenoid cystic carcinoma, and acinic cystic carcinoma. Salivary gland tumor models would be extremely beneficial in discovering and characterizing new biomarkers for diagnostic use.
Modern Implementation of 3D Culture Systems
Salivary diagnostic platforms can be considered from multiple perspectives of the complete native gland system. Salivary secretions (i.e., saliva) may contain biomarkers that reflect an organism’s health. In vivo interrogation of a living tissue (through live microscopy or other means) may yield information on tissue structure or permit the targeted collection of cells or biological materials. In vitro culture of salivary cell lines enables researchers to observe cell structure and behavior and assess cell response (e.g., protein/gene expression). Beyond all of these existing methods, 3D culture of salivary cells and tissues enables in situ access to the developing and functioning gland while promising a richer level of understanding of gland responses to environmental changes, e.g., drugs, altered extracellular matrix, or introduction of genes for targeted expression of necessary proteins.
The accuracy of salivary gland models relies on the replication of necessary minimal features of each element of the native gland. Cells can be cultured in 3D spheroids, but will require some surrogate of the ECM in order to receive and respond to the same mechanical and biological cues experienced by an intact gland. In the same way, an ECM system with high fidelity to native tissue requires a cell line (or primary cells) with accurate representation of in vivo phenotypes and likely also requires a mixture of multiple cell types, in proper ratio and geometry.
These same issues are fundamental to the broader field of tissue engineering and regenerative medicine, and advances in these fields can benefit similar efforts at advancing 3D salivary diagnostics models. The following sections will consider each element of the traditional tissue engineering model (ECM support and cell source), with a focus on their use in vitro.
Hydrogels Versus Microporous Scaffolds
Numerous reviews have documented the historical path of today’s tissue engineering [105–109]. As researchers from the polymer and plastic communities intersected with colleagues in the clinical and biological disciplines, polymer engineers sought to mimic native ECM structures using existing polymeric materials. The key advances in this effort were the transitions from biocompatible to bioinert to biodegradable materials. Poly(α-esters), such as poly(glycolic acid) (PGA) and poly(lactic acid) (PLA) and their copolymers (PLGA), were primary entry points for nascent tissue engineers, since these offered physical properties similar to other common thermoplastics, but with degradation profiles that enabled the potential reentry of soluble hydrolysis products back into the cell cycle. The relative strength of these degradable plastics allowed them to be implemented in a manner similar to other thermoplastics; i.e., they could be extruded into fibers and woven into fabrics, as in Fig. 8.2, cast from organic solvent into complex structures or molded with sacrificial microparticles (“porogens”) into microporous assemblies. These structures continue to find use in numerous tissue engineering applications, particularly in the regeneration of load-bearing musculoskeletal systems or in tissues with high native structural requirements.
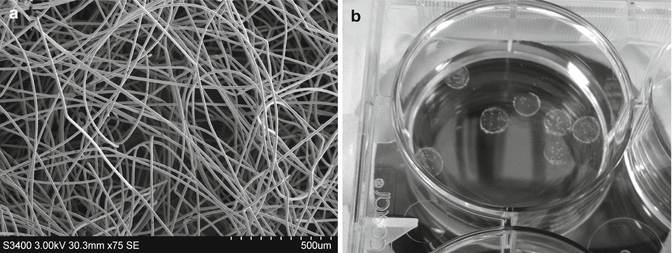
Fig. 8.2
Biomaterial supports for 3D cell culture. (a) Nonwoven PGA microfiber scaffold, imaged by scanning electron microscopy. (b) Hyaluronic acid polymer hydrogels, cast into discs 6 mm in diameter
However, a significant issue with these microporous scaffolds is that the polymer only mimics the concept of a 3D ECM, but not necessarily the mechanical, biological, or diffusive properties of the native 3D matrix. Polymer matrices such as PGA and PLA are orders of magnitude stiffer than the native ECM and prohibit the diffusion of important cell metabolites and signaling molecules—everything from oxygen to growth factors—across their boundary. Investigators soon discovered that interconnected pores were required for cell seeding and growth, since this would be the only path to allow cell-cell contact with other pores and (in the absence of vascularization) sufficient nutrient penetration into the core of a scaffold. While microporous scaffolds resemble 3D tissue structure, in many ways cells on these scaffolds experience a compact 2D structure, on an unnaturally stiff substrate. However, investigators continue to invent novel applications for these materials in modeling salivary structure and influencing salivary cell assembly, such as a recent description of micropatterned “craters” used to encourage acinar cell arrangement [110]. Electrospun solutions of PLGA in hexafluoroisopropanol (HFIP) yielded nanofibers in the range of 50–150 nm, which could be collected as a mat and molded to reproduce the pattern of microscale craters. These nanofibers, on the order of 100× smaller than conventional PGA microfibers, can better reproduce the native ECM and yield an improved application of this well-characterized biodegradable polymer.
In contrast, the modern hydrogel system employs an alternate 3D concept by using inherently hydrophilic molecules (either polymeric or small molecules) that can be triggered to surround and encapsulate cells fully, within a cross-linked network swollen with water. The triggers for gelation may be reversible (such as the use of Ca2+, in the case of alginates and self-assembling peptides) or permanent covalent cross-links (through complementary reactive groups on adjacent polymer chains or through the use of a photocatalyst to initiate covalent cross-linking among chains). Microporous scaffolds employ pore sizes on the scale of 50–500 μm, to enable cell penetration during seeding; in contrast, hydrogel pore sizes are nearly three orders of magnitude lower in typical use—small enough that the diffusion of even some large proteins may be retarded.
For the replication of soft tissues, hydrogel scaffolds present a contrasting replication of two major elements of the native ECM:
-
Mechanical PropertiesNumerous recent studies have demonstrated that a scaffold’s mechanical properties are paramount to influencing the phenotype of the cells encapsulated within [111]. Cells sense and respond to their physical environment through multiple mechanisms, many of which are still being discovered. The assembly of integrin receptors into focal adhesions is a classic phenomenon of 2D culture on glass or tissue culture polystyrene substrates, and that behavior translates to cell attachment onto PLA/PGA microporous scaffolds. Such focal adhesions convey substrate stiffness through the cytoskeletal network, and additional intracellular signaling occurs through the proteins assembled at a focal adhesion structure. However, this behavior changes significantly when cells are fully encapsulated in a 3D hydrogel matrix [112] since moduli in the range of 100–1,000 Pa are most common for non-muscle soft tissues. The reduced stiffness of hydrogel matrices alters the size, frequency, and localization of focal adhesion plaques, as well as the arrangement of actin filaments, and these impact cell phenotype. Numerous reports have linked the abnormal expression of signaling proteins and cytoskeletal focal adhesions to a nonphysiological culture environment [113–115].Swift et al. have recently examined a range of tissues, spanning nervous tissue to muscle to osteochondral tissues, and demonstrated a shift in the ratio of lamin isoforms (specifically lamin A to lamin B ratios) as a function of ECM modulus [116]. Lamins are present in the nuclear membrane of each cell, and the balance of isotypes influences nuclear membrane stiffness. As described by Swift, however, these proteins also affect transcription and enhance the lineage direction of cells. This example is only the most recent in a series of significant publications by Discher and coworkers, regarding the impact of substrate modulus on cell differentiation and its correlation to cytoskeletal components and nuclear mechanics [111, 117, 118].
-
Diffusion of Soluble ComponentsThe diffusion of small molecules and growth factors through a tissue is regulated largely by the molecule’s size, its molecular characteristics (e.g., hydrophilicity, charge), and its interaction with other ECM components. While such molecules do not pass through tissues unimpeded, their diffusion through fields of ECM components is possible due to the aqueous nature of body tissues. (Cartilage, a nearly avascular tissue, is an example of how nutrients and metabolites can be delivered via diffusion in aqueous networks.) Hydrogels and microporous scaffolds represent opposite extremes of this model: While microporous scaffolds with interconnected pores permit fluid flow across open interpore channels, cells may still remain isolated by largely impermeable walls that prevent contact between cells on opposite sides. Conversely, the open, swollen network structure of a hydrogel enables the diffusion of soluble molecules throughout the system, which then permits paracrine interaction among encapsulated cells. At low cell densities, this open network may reflect the opposite extreme, representing the lowest possible inhibition of nutrient diffusion. Under typical use, however, the hydrogel model best mimics the native ECM structure and function.
A third element of the native ECM has been implemented to varying degrees on hydrogel and macroporous scaffolds:
-
Biologically Relevant Native EpitopesThis group represents the set of minimal ECM features that have been identified as crucial to cell survival, migration, organization, or differentiation. Generally, these features are categorized as:
-
ECM Adhesion Sequences: Minimal peptide sequences, particularly the RGDS sequence identified from fibronectin [119, 120], have been shown to promote integrin-based adhesion, which is crucial for many tissue-engineered model systems. Although intact adhesion-promoting proteins will quickly adsorb onto most scaffolds from fetal bovine serum in cell culture media, numerous applications require the deliberate integration of these sequences into or onto a scaffold.
-
Cell–Degradable Sequences: Cell migration through the native ECM is mediated by the cell’s toolbox of proteases and glycosidases. For in vitro applications using a non-proteinaceous scaffold material, investigators requiring cell migration must “build in” labile sites that are susceptible to the cell’s inherent degradative enzymes. Matrix metalloproteinases (MMPs) have been well characterized, and numerous minimal substrate sequences have been identified (e.g., GPQG↓IAGQ, derived from collagen I) [121], which can be synthesized chemically and integrated into or onto a biomaterial. A classic example of synthetic PEG hydrogels, rendered susceptible to MMP degradation through the incorporation of an MMP substrate, was described by Lutolf et al. [122].
-
Polysaccharides: This diverse class of macromolecules often influences cell behavior indirectly in the ECM, by either enabling or resisting the retention of proteins. Heparan sulfate oligomers, for example, may serve as depots for potent heparin-binding growth factors; conversely, heparin may be tethered to biomaterial surfaces to reduce clotting.
-
Cell Adhesion Molecule Mimics: Many cells rely on cell-cell adhesion molecules (CAMs) for orientation and guidance, rather than cell-ECM interactions. Recombinant versions of these proteins or their fragments can be covalently incorporated into/onto 3D scaffolds to encourage the survival or phenotypic arrangement of such cells (e.g., ephA-ephrinA signaling in pancreatic β-cells [123] or ECM components such as perlecan fragments that may resemble CAMs and influence cell behavior in similar ways [6]).
-
The notable difference between the incorporation of biologically relevant epitopes in microporous scaffolds and hydrogels is a matter of “on” and “in.” Such biomolecules can be covalently tethered onto the surface of microporous scaffolds through chemical modification of the scaffold surface and thus are typically present as a monolayer or a thin surface layer. Conversely, biorelevant peptides, proteins, and proteoglycans can be incorporated throughout a hydrogel, either through covalent attachment in the initial gelation step or simple diffusion into the established network.
A related consequence of these contrasting paradigms is their varying suitability for visual analysis by conventional techniques of confocal microscopy and histologic preparation. In general, hydrogels are sufficiently transparent that encapsulated cells can be observed via light microscopy (phase imaging or DIC), or fluorescence imaging of small molecules (live/dead stains, cell trackers, organelle tracers, etc.) and fluorescent proteins (e.g., GFP fusion proteins), or immunofluorescence, enabling real-time observation of cell response to culture conditions. Confocal microscopy methods enable the detailed reconstruction of cell features in 3D over several hundred microns. Conversely, these methods are difficult or impossible for most microporous scaffolds, since light cannot easily penetrate many common polymers or diffract so heavily that detailed imaging of cellular arrangement or phenotype beyond the exterior layer of a scaffold is obscured. Histologic methods are similarly affected by the scaffold composition; hydrogels with a high water content and low cell density or ECM density are not amenable to standard paraffin embedding, but can be adapted to cryoembedding, microtoming, and staining methods. Microporous polymer scaffolds can be more difficult to adapt to these methods, since standard paraffin embedding reagents may dissolve the most commonly used polymers, and cryomicrotome methods may be more difficult with the higher stiffness of these scaffold materials. For our present interest in salivary diagnostic techniques, hydrogels are generally more amenable to assessment by conventional imaging methods (discussed in more detail later in this chapter).
Currently Available Biomaterials
Many synthetic materials may be modified with adhesive proteins to encourage cell attachment or promote a specific phenotype within a hydrogel. Collagen and laminin are common choices for this, and some commercial hydrogel kits (e.g., the HyStem series of hyaluronan-based hydrogels) include variations that incorporate proteins toward this purpose. It would be expected that such proteins would alter cell phenotype, given the presence of laminins and collagen IV within the basement membrane surrounding acinar structures. Conversely, other adhesive proteins contain binding sites that could promote a migratory phenotype, rather than a stabilized acinar structure. The details of the protein preparation are crucial, since covalent cross-linking of proteins or peptide fragments into another polymer network often requires chemical modification, and the degree of functionalization, nature of the cross-link chemistry, and steric accessibility will all influence the eventual consistency of the matrix structure. Similarly, specific processing details of these proteins can influence their biological function and their purity. One example of the influencing factors that may affect protein performance from biological perspective was described by Dhimolea et al. [124]. Collagen sourced from two different species (rat and bovine) performed differently in influencing mammary epithelial cell organization and phenotype. This could be attributed to differences in the species-specific sequence of the protein (which should be minimal, as many collagen structures are highly preserved), which could lead to changes in bundle formation, or differences in the protein processing steps, which could also lead to variation in collagen fibril isolation, and reassembly in the final hydrogel. Variations in collagen organization can lead to variations in matrix stiffness, which then influences cell behavior, as described previously.
The biomaterial literature describes numerous laboratories that synthesize traditional and/or custom matrices for the 3D in vitro culture of multiple cell types. Bulk biodegradable polymers, such as PGA, PLA, PLGA, poly(ε-caprolactone), and others, are also available commercially through chemical vendors (e.g., Sigma-Aldrich, Polysciences), and methods for creating porogen-leaching microporous scaffolds (most often manufactured using sodium chloride crystals, sieved to a selected size range, as a porogen) are well described in the literature. Similarly, multiple literature reviews describe methods for electrospinning these polymers from solutions in organic solvents into fibrous mats with fine micro- and nanoscale textures. Each of these methods for scaffold synthesis has matured over the past decade, along with complementary and necessary methods for characterization, sterilization, and seeding with cells, and all can be considered available and accessible to any laboratory.
While the majority of matrices used in tissue-engineered applications are polymeric, a unique class of biomaterial considered in this study is the self-assembling peptide (SAP) [125]. SAPs are small peptides that are preprogrammed to assemble into multimolecular hydrogel structures, with one or more levels of spatial organization, usually triggered by temperature, pH, or an ionic gradient. Self-assembled hydrogels often have weaker mechanical properties than polymeric gels, but are well within the desired range for cell encapsulation.
The commercial options for all of these are increasing and offer the benefits of quality control and specified parameters appropriate to each scaffold type (e.g., degradation rate, pore size distribution, cross-linking rate, endotoxin, sterility, etc.). A survey of current commercial options includes the following:
-
Commercial Microporous Scaffolds:
-
BIOFELT (Biomedical Structures)—PLA, PGA, and PLGA woven (i.e., resembling a braided fabric) and nonwoven (i.e., random, resembling felt) scaffolds. The choice of polymer will dictate the degradation profile of the scaffold. One of the most common examples of these scaffolds is a nonwoven PGA matrix, with fibers 10–15 μm in diameter and pore sizes >100 μm in size.
-
Alvetex (reinnervate)—polystyrene microporous scaffold. Although not biodegradable, these microporous scaffolds have an exceptionally open structure with high interpore communication.
-
-
Commercial Hydrogel Kits:
-
Matrigel (BD Biosciences)/Cultrex (Trevigen)—One of the most widely used hydrogel matrices for modeling cell behavior in 3D culture, basement membrane extract (BME), is sold under the trade names of Matrigel and Cultrex as a solution of basement membrane proteins (largely comprising laminin, collagen IV, perlecan, and numerous potent growth factors) isolated from Engelbreth-Holm-Swarm (EHS) mouse sarcoma cells. Multiple formats and preparations of BME are available, including some with reduced growth factor content. While soluble at cold temperatures, BME proteins aggregate irreversibly to form a solid hydrogel structure at room temperature. Most cells adhere readily to BME-coated surfaces and gels.
-
Collagen I (multiple vendors)—Most frequently derived from rat tail, collagen I gels have seen frequent use as matrices for cell culture, but their applicability to salivary gland models may be limited. Excess collagen I is often associated with specific tissue systems and/or a scar and repair phenotype in a wound response. These cues, along with the slow but persistent contraction of collagen gels, may induce an undesired phenotype in cells that prefer a more compliant tissue.
-
HyStem—hyaluronic acid (HA)-derived hydrogels. HA from bacterial sources is thiolated at free carboxyl groups along the polymer and cross-linked with PEG-diacrylate. The thiol-acrylate “click” chemistry proceeds quickly via a Michael addition mechanism, with no by-products and minimal reactivity with the surrounding biological components. Variations of HyStem include a thiolated gelatin component (HyStem-C) that can promote cell adhesion and a thiolated heparin component (HyStem-HP) that can retain heparin-binding growth factors for delayed release. The HyStem system’s relevance is due to the pervasive presence of HA in the human body and the expression of HA receptors (RHAMM/CD168 and CD44) by many cells.
-
QGel MT 3D Matrix (QGel)—PEG-based system, also based on a Michael addition for gelation within 10–20 min of gelation. PEG is an unnatural polymer that is biocompatible, but resists protein adsorption and is nondegradable by most native cell enzymes. QGel systems incorporate pendant RGD moieties, to enable cell adhesion, and integral MMP-degradable peptides, to allow for cell migration through the hydrogel.
-
AlgiMatrix (Life Technologies)—alginate hydrogel derived from seaweed. Alginates can be purchased in this format, or in bulk, and gelled quickly in cationic solutions (e.g., CaCl2). Encapsulated cells can be quickly released from the matrix (e.g., with EDTA). Alginates provide no sites for integrin-based adhesion by cells and are not easily degraded by cells. However, these systems have found great utility for 3D encapsulation and culture of many cell types (e.g., retention of chondrocyte phenotype, preservation of oocyte viability) and have been modified to include RGD sequences.
-
MAPtrix (Kollodis)—ECM derived from recombinant mussel adhesive proteins (containing an 18 % lysine content), cross-linked with multiarm PEG-succinimide.
-
PuraMatrix (3D Matrix Medical Technology)—unlike the other polymeric hydrogel matrices, PuraMatrix is an example of a self-assembling peptide system. The sequences of small peptides are engineered to enable their assembly upon trigger with ionic or pH gradients and form nanoscale structures that entangle to form a hydrogel structure.
-
The contrasting advantages and disadvantages of the aforementioned matrices—particularly the hydrogel systems—depend on the application. For researchers interested in cell encapsulation with an eventual application in regenerative medicine (i.e., implantation in humans), the animal-derived hydrogel systems (Matrigel/Cultrex, collagen I) are unsuitable, as these would not receive FDA approval for implantation. However, for salivary diagnostic models, particularly in vitro use, such protein-based systems may induce the optimal cell response. Researchers may consider some of the other listed materials to meet other criteria, such as cost, ease of use, or more granular control over hydrogel composition. In general, a wide range of biomaterial platforms are available, and only a small subset have been explored in the context of preserving, reconstituting, or analyzing salivary gland structure or function.
In Vitro and In Vivo Imaging
Advances in optical imaging hardware and software have increased the depth to which we can explore salivary gland physiology in situ and our ability to resolve objects, even down to the molecular scale. Improvements in fluorescent probe technology enable the detection of specific proteins, molecular processes, and cellular activation over extended length and timescales. Three-dimensional in vitro models in transparent hydrogels benefit from advances in both of these fields, particularly due to the longer working distances and multicellular assemblies that are characteristic of these cultures.
Imaging Techniques
Epifluorescence Microscopy
Epifluorescence microscopy uses a mercury or xenon arc lamp to illuminate the specimen or excite fluorophores within a specimen in the ultraviolet (UV) to visible light spectrum. The light is directed to and from the specimen by a series of mirrors and bandpass filters to allow photons of specific wavelengths to reach the eye port and/or camera for detection. Specific fluorophores targeting proteins of interest within a prepared specimen may be excited to emit photons that are detected by the epifluorescence microscope. The fluorescing areas will appear bright against the dark background and may be quantified using post-processing imaging software to determine protein expression and distribution within the specimen. Excited fluorophores undergo a “Stokes shift,” in which the emitted light occurs at a longer wavelength (i.e., lower energy) than that of the incident light. Detection filters are therefore set to block the lower excitation wavelength and solely detect the longer emitted wavelength. Epifluorescence microscopy has been a powerful tool for determining protein distributions and live-2D visualization of the onset intercellular signaling using calcium indicators, voltage sensitive dyes, and small molecule fluorescent dye transfer in vitro and in vivo [126].
Confocal Microscopy
Confocal microscopy is an optical imaging technique that utilizes focused scanning beams of light across a specimen in the UV to visible light spectrum. Lasers are a more powerful light source and are used to excite molecular tracers or targeting fluorophores within a specimen. Emitted photons are collected and detected by sensitive photomultiplier tubes (PMTs) to generate an image. Light scatter within a specimen at these shorter wavelengths is proportionate to the thickness and translucence of the specimen, so it is important to consider these limitations when resolving anatomical or subcellular structures in hydrogel and tissue specimens. Compared to conventional epifluorescence microscopy, spatial resolution in confocal microscopy is considerably higher because of focused light and an adjustable pin hole to reduce photon scatter prior to detection. Sharp image slices may be captured through a specimen at intervals and reconstructed in 3D and are optimal for colocalization studies.
Confocal microscopy may be used to image 2D and 3D cell culture models as well as live animal models where lateral and axial resolution are determined by the excitation wavelength λ ex, numerical aperture NA of the objective lens, and the refractive index n of the immersion medium [127] (see Eqs. 8.1 and 8.2). The refractive index n for air, water, and oil is 1.00, 1.33, and 1.52, respectively.
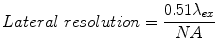
(8.1)

(8.2)
Motion artifacts from animal respiration and heartbeat pose technical challenges in live imaging but may be overcome [128]. Also, phototoxicity and photobleaching may affect the integrity of the tissue as well as the intensity of fluorophores when imaging over time to create challenges when quantifying responses with respect to fluorescence intensity. Although presented with these challenges, confocal microscopy is still ideal where penetration depths are uniform to the surface to ensure optimal conditions and resolution of subcellular structures. Confocal microscopy has more recently been used to quantitatively determine the effectiveness of mouthwash on the oral flora [129].
Multiphoton Microscopy
This technology is based on nonlinear emissions and can image microstructures and intercellular processes beneath the skin. In this method, a fluorophore with a maximum excitation wavelength of λex can be excited by the simultaneous “additive” absorption of two photons, each of a wavelength 2λex (or corresponding energy E/2). For example, a fluorophore that normally excites maximally at λex ~400 nm and has a maximum emission at λem ~550 nm can be excited by two coincident photons of λ ~800 nm each. (The excited fluor would still emit maximally at λem ~550 nm.) A much higher laser density is necessary in order to achieve this coordinated photon absorption, and specialized lasers (“pulsed” or “mode-locked”) are required to obtain this high flux.
Although multiphoton microscopy offers no improvement in resolution over conventional confocal microscopy, the method does confer two other significant advantages. First, the use of 2λex wavelengths in the IR range enhances laser penetration into the specimen of interest, since these longer wavelengths are less commonly absorbed by biological tissues. Secondly, the physical nature of multiphoton excitation relies not only on the exact temporal coincidence of the two low-energy photons but also a correspondingly high spatial focus. To understand the relevance of this idea, consider that, under conventional epifluorescence methods, an entire specimen is illuminated under the filtered light of a xenon/mercury lamp and the fluorescing moieties within a given depth of the focal plane are imaged. Because of this imaging configuration, the entire specimen may be gradually photobleached due to continual exposure to excitation wavelengths. Confocal microscopy offers the advantage of a scanning laser, with a reduced excitation cross section and dwell time, but it still exposes an entire specimen throughout its depth to extended excitation. In contrast to both of these methods, multiphoton excitation occurs solely at a precise point in space within the focal plane, where photon density is sufficiently high and coherent. All other laser light above and below the focal plane has insufficient coincidence to excite any fluorophores or induce any damage from photobleaching. These properties enable extended live imaging of thick in vitro samples or in vivo carriers with far less possibility of specimen damage.
Imaging intact tissue enables researchers to study biological processes in their native microenvironments and to extract physiologically relevant information. It has allowed researchers to image neuronal activity in brain tissue [130], macrophage motility during tumor cell invasion [131], secretory granule dynamics in salivary gland tissue [132], and Cl− transport in salivary epithelium [133]. Two-photon excitation has been used to “uncage” bioactive compounds to visualize real-time intercellular communication in monolayers of cultured mouse skeletal muscle cells [134] and neuronal synaptic transmission in rat hippocampal slices [135]. Currently, two-photon microscopy is used to study various aspects of cell biology with cellular resolution in live mice under anesthesia [128] as well as in freely mobile rats [136].
Multiphoton microscopy allows for the observation of vascular and neuronal integration of seeded hydrogels implanted in the salivary bed. Longitudinal observations would be useful to track the progression of biological integration. Effects of pharmacological and mechanical perturbations on the system aimed to accelerate and encourage structural incorporation may also be observed with the native microenvironment.
Intensity-Based Imaging Techniques
A multitude of techniques take advantage of the consequential photobleaching of fluorophores in high-resolution, intensity-based imaging to study intercellular and subcellular molecular dynamics. Two well-established techniques are fluorescence recovery after photobleaching (FRAP) and fluorescence loss in photobleaching (FLIP). Focal regions within a specimen with fluorescently tagged molecules may be photobleached and imaged over time to determine molecular diffusion constants, protein synthesis and turnover rates, and subcellular connectivity.
Complementary techniques include fluorescence resonance energy transfer (FRET) and fluorescence correlation spectroscopy (FCS), which are used to observe interactions at the molecular scale. In FRET, if two fluorophores are within a very short distance of each other (usually <5 nm), some fraction of the excitation energy needed to excite the shorter wavelength fluorophore is transferred to excite the longer wavelength fluorophore through dipole-dipole coupling [137]. FRET donor-acceptor pairs are particularly sensitive to intermolecular distances, with FRET efficiency scaling on the order of R6 (where R is the distance between fluors). A typical application of this technology is in the assessment of protein-protein interactions, either intramolecular (i.e., protein folding) or intermolecular. Individual proteins may be labeled with synthetic probes, or generated as fusion proteins, and assessed at the donor excitation wavelength. In theory, the proteins interact closely if the acceptor emission wavelength is observed and do not interact closely if the donor emission wavelength is observed. In practice, however, mixed populations of both wavelengths may be present, depending on the kinetics and stability of the system in question, and researchers must use population averaging and photobleaching controls to clearly delineate these effects. A FRET-based biosensor, Chameleon, uses the Ca2+ binding sites of calmodulin to link two fluorophores—yellow fluorescent protein (YFP) and cyan fluorescent protein (CFP)—so when Ca2+ binds to the construct, the binding domain folds to cause FRET. FRET has been used in live drosophila salivary gland to investigate caspase-mediated cell death during development [138]. FCS has been proposed as a less expensive and laborious method of detecting biomarkers in solution [139]. Another technique widely used to observe plasma membrane dynamics within 200 nm of the surface can be accomplished by total internal reflection fluorescence (TIRF) to observe cell adhesion [140] and receptor binding at the surface of the cell.
Small Animal Imaging
The great challenge of imaging physiological processes within small animals is overcoming the many biomolecular obstacles that absorb, distort, or scatter signals from the tissues, cells, or molecules of interest. This single challenge is additionally compounded for living animals under long-term observation. Noninvasive, nondestructive imaging can restrict the extent and quality of available information from a single animal, despite the investigator’s goal of obtaining the greatest amount of data possible. High contrast therefore remains the ultimate goal for any imaging method. Luciferase-based (luminescent) reporter constructs continue to be popular for this reason, because, despite a comparably lower signal than many fluorescent probes, luminescent reporters are observed over a substantially lower background, yielding a greater signal-to-noise ratio (S/N). The advent of high-resolution, low-noise, cooled CCD detectors has yielded significant improvements in signal detection and resolution. As an example, longitudinal evaluation of cell-seeded 3D scaffolds and hydrogel implants in the rodent salivary bed can be conducted using small animal imaging systems. Commercial examples include the multimodal IVIS® (Thermo Perkin-Elmer, formerly Caliper) and In-Vivo (Bruker, formerly Carestream/Kodak) imagers, which combine sensitive detectors for fluorescence, luminescence, and often X-ray imaging.
Selection of fluorescent and luminescent reporter probes (as described further in the next section) is critical and must provide appropriate image S/N ratio. In another example, optical-resolution photoacoustic microscopy (OR-PAM) has been employed in vascular imaging to provide structural information about vessel density and vascular permeability and integrity [141
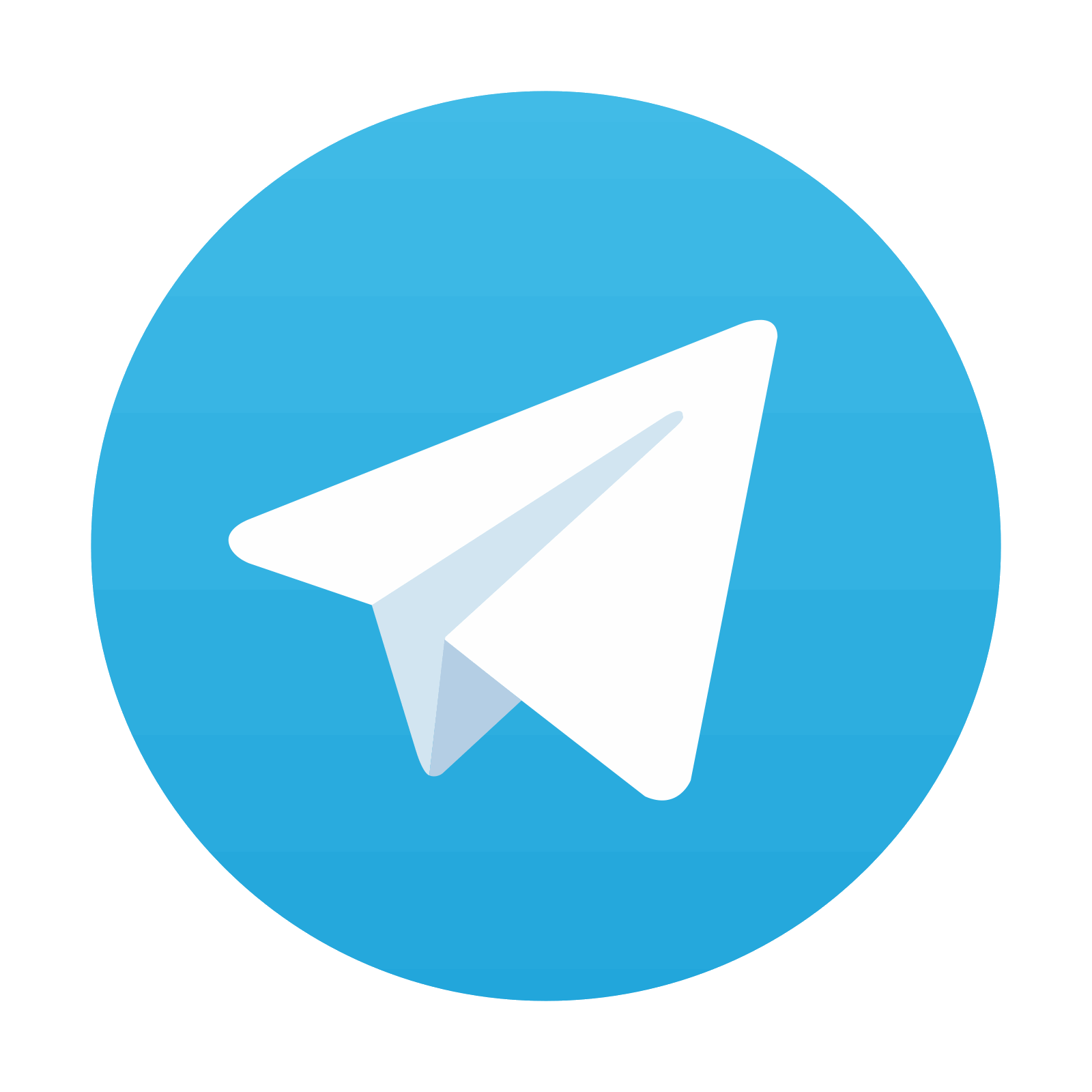
Stay updated, free dental videos. Join our Telegram channel

VIDEdental - Online dental courses
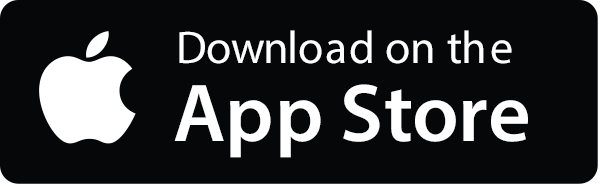
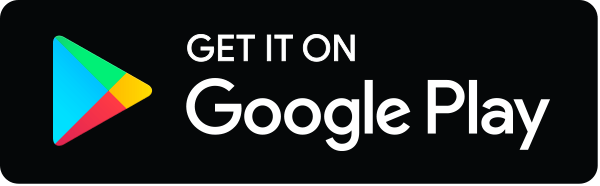