3
Polymer Materials for Oral and Craniofacial Tissue Engineering
Introduction
The oral and maxillofacial regions are complex areas since they are composed of different tissues. These regions can be affected by abroad range of pathologies, congenital defects, oncologic resection, trauma and infections (Susarla et al. 2011). The current strategies involve the use of allogenic, xenogeneic and autogenic grafts (Wang et al. 2005). However, there are drawbacks regarding the graft rejection, transmission of diseases and infection causing regeneration failure. Moreover, the complexity of cranio-maxillofacial tissues is a challenge due to the interactions between different types of tissues, including epithelium, mineralized and non-mineralized connective tissues (Bartold et al. 2000; Aurrekoetxea et al. 2015). Researchers focused on the study of new strategies based on the basic principle of tissue engineering—which means the use of cells, scaffolds and bioactive molecules to regenerate damaged tissues.
In this scheme, the use of stem cells derived from different sources alone or in combination with growth factors or gene therapy plays an essential role in tissue engineering because stem cells are self-renewable and can differentiate into different cell lines (Horst et al. 2012). Meanwhile, the scaffolds should provide a porous and stable structure to mimic native ECM and allow cells to synthesize their own matrix (Kim et al. 2016; Chanes-Cuevas et al. 2018). The proper biological, physical and chemical characteristics of scaffolds have led researchers to develop bio-inspired devices with tunable morphology and bioactivity using different technologies and materials.
For this purpose, biodegradable materials have been studied as a promising approach to design biomaterials for tissue engineering. Polymers have been extensively proposed for their use in biomedical applications due to their biodegradability and biocompatibility (Ozdil and Aydin 2014). Polymers can be classified into two major groups depending on their source: natural and synthetic polymers respectively. Polymers have been extensively used as biomaterials for different applications, i.e., wound healing, bone, nerve, tendon, muscle, cartilage and cardiac tissue engineering. A wide variety of biomaterials have been used for craniofacial and alveolar bone, gingival tissue, periodontal ligament, dental pulp, cementum, dentin and drug delivery systems (Gupte and Ma 2012; Kim et al. 2014).
Synthetic Polymers
Biocompatible synthetic polymers are promising materials with tunable properties in terms of degradation rate, biocompatibility and mechanical properties. The most commonly used synthetic degradable polymers for tissue engineering and drug delivery are mainly aliphatic polyesters including Poly(Lactic Acid) (PLA), Poly(Glycolic Acid) (PGA), Poly(Caprolactone) (PCL) and their copolymers. These polymers are approved by the U.S. Food and Drug Administration (FDA) for clinical applications (Table 3.1).
PLA is a biodegradable, bioabsolbable and thermoplastic polyester, with excellent mechanical properties, obtained from renewable resources (Gupta et al. 2007). The monomer and acid lactic, have two optical isomers, L- and D-lactic acid, and PLA has three stereoisomers, poly(L-lactic acid) (PLLA), poly(D-lactic acid) (PDLLA) and poly(DL-lactic acid). The isomer L-lactic acid is found in living organisms and is the main fraction of PLA obtained from renewable sources (Fukushima and Kimura 2008). PLLA has gained attention for its use in tissue engineering due to its excellent biocompatibility and mechanical properties. PLA can be degraded by non-enzymatic hydrolysis into lactic acid, a bio-product which can be metabolized by normal cells. Due to the crystalline nature, PLA is a long-time degradable material, that can form crystalline fragments during the degradation process, causing an inflammatory response. That is why the combination of L- and D-isomer could be used to control the degradation time, crystallinity and melting point (Carrasco et al. 2010; Lopes et al. 2014; Santoro et al. 2016a).
PGA is characterized by low solubility in most organic solvents, as a function of the molecular weight. However, as molecular weight is too low, the solubility increases but the mechanical properties can be lost (Ma and Langer 1995). PGA was first used for absorbable sutures, due to its relative hydrophilicity nature which allows its rapid degradation and loss of its mechanical integrity in around 2-3 weeks (Ma and Langer 1995). PLA and PGA are biocompatible materials, with controllable degradation rate depending on chemical structure, molecular weight and crystallinity of polymers (Pamula et al. 2001). In order to extend the applicative use of PGA, it has been used to form co-polymer with PLA, a more hydrophobic polymer, suitable to regulate the degradation of PGA. Poly(lactic acid-co-glycolic acid) (PLGA) may combine the advantages of both polymers, by modulating the co-polymer composition. For instance, high fractions of glycolic acid make the PLGA degradation faster, higher portions of PLA make it stiffer (Makadia and Siegel 2011). PLGA has been used for tissue engineering scaffolds and particularly to fabricate nanoparticles for drug delivery systems, due to the broad range of the degradation time (Moioli et al. 2007; Makadia and Siegel 2011; Danhier et al. 2012).
PCL is a semi-crystalline polymer that can be easily processed and dissolved with organic solvents. PCL is a biocompatible polymer with a slow degradation rate driven by hydrolysis of ester linkages, that allows addressing its use to a long regeneration process as in the case of the bone (Lam et al. 2009; Xu et al. 2015). PCL has also been frequently proposed in the form of a drug carrier, being able to uniformly distribute bioactive molecules and drugs into the matrix, and releasing them by a controlled degradation mechanism for sustained delivery (Luong-Van et al. 2006). In order to overcome some limitations of PCL, due to its hydrophobic properties, PCL was frequently blended with hydrophilic polymer phases, such as collagen, gelatin, keratin, elastin, silk among others suitable to enhance cell-material interactions (Guarino et al. 2011; Zhang et al. 2015; Aguirre-Chagala et al. 2017; Cruz-Maya et al. 2019).
Table 3.1. Principal synthetic polymers for oral and craniofacial tissue regeneration.
Synthetic polymers | Application | Reference |
Poly(s-caprolactone) (PCL) | Alveolar bone, GBR, periodontal ligament, drug delivery | (Cirillo et al. 2014; Guarino et al. 2017b; Cruz-Maya et al. 2019) |
Poly(lactic acid) (PLA) | Bone, periodontal ligament | (Suarez-Franco et al. 2018; Vazquez-Vazquez et al. 2019) |
Poly(glycolic acid) (PGA) | Periodontal ligament, pulp regeneration | (Mooney et al. 1996) |
Natural Polymers
The increased interest to reproduce the extracellular microenvironment to promote tissue regeneration has led to developing materials based on natural polymers (Cmz-Maya et al. 2018). Among them, proteins—including collagen, gelatin, fibrin, elastin, keratin, silk, zein—and polysaccharides such as chitosan, hyaluronic acid, alginate; and polynucleotides have been differently used for the design of materials in oral tissue engineering (Table 3.2) (Smith et al. 2016).
Collagen is the most abundant protein in the human body and the main compound of ECM. Collagen is a triple helix protein, characterized by a repetitive sequence of amino acids, glycine, proline, and hydroxyproline (Sherman et al. 2015). Currently, it can be identified by more than 20 types of collagen types. Collagen type I is the most abundant in nature followed by collagen type III (Parenteau-Bareil et al. 2010). Collagen and its denaturized form, gelatin, have been intensely studied for their use in tissue engineering because of their excellent biocompatibility and the presence of RGD sequences to promote integrin-mediated cell adhesion (Parenteau-Bareil et al. 2010). To improve the properties, collagen has been blended with other polymers for their use in periodontal tissue regeneration. Gelatin is a protein derived from the partial hydrolysis of collagen and depending on the extraction and manufacturing method there are type A, acidic treatment, and type B, alkaline treatment (Aldana and Abraham 2016). Gelatin has several advantages for tissue regeneration, as RGD-like sequences to promote cell adhesion, biocompatibility, and because it is a denatured form of collagen, gelatin is less antigenic (Su and Wang 2015).
Elastin is the second most common protein in ECM, being responsible for the elasticity and resilience of tissues. Elastin is characterized by its hydrophobic nature due to several amino acids in its composition. The precursor of elastin is tropoelastin, characterized by hydrophobic domains, composed basically by alanine, proline, valine, leucine, isoleucine and glycine conferring the elasticity of protein (Rodriguez-Cabello et al. 2018). The elastin lacks RGD motifs, however, it is recognized by integrins, as integrin αvβ5 allowing integrin-mediated cell adhesion (Lee et al. 2014). Elastin has been widely studied to create biomaterials for applications where elasticity is required, as wound dressing, arterial or vascular applications, lung and cartilage (Grover et al. 2012; Milleret et al. 2012; Minardi et al. 2016).
Table 3.2. Principal natural polymers for oral and craniofacial tissue regeneration.
Natural polymers | Application | Reference |
Collagen and gelatin | Alveolar and bone, GBR, periodontal ligament | (Tal et al. 1996; Stoecklin-Wasmer et al. 2013) |
Elastin | Bone, periodontal ligament, salivary glands | (Foraida et al. 2017) |
Silk fibroin and sencin | Periodontal ligament, pulp regeneration, maxillofacial bone defects, implant osseointegation | (Mooney et al. 1996; Sangkert et al. 2017) |
Keratin | Periodontal ligament, coating of transmucosal implants, drug release | (Ferraris et al. 2017; 2018; Cruz-Maya et al. 2019) |
Zein | Bone, periodontal regeneration, drug delivery | (Zhou et al. 2014; Yang et al. 2017; Bonadies et al. 2019) |
Polysaccarides (Chitosan, hyaluronic acid, alginate, cellulose) | Gingiva augmentation, dental pulp regeneration, bone regeneration, drug delivery, antibacterial activity, periodontal regeneration | (Becker et al. 2010; Inuyama et al. 2010; Moshaverinia et al. 2012; Zhou et al. 2014; 2017; Chamieh et al. 2016; Ni et al. 2019) |
Silk fibroin is a natural fiber protein that has gained attention for biomedical applications requiring an improvement of mechanical properties (i.e., flexibility and high tensile strength). Silk fibers extracted from domesticated silkworm Bombix mori (B. mori) are the best characterized. The amino acid composition of silk consists of glycine, alanine, serine (Vepari and Kaplan 2007; Ma et al. 2018). Silk is composed of a filament core coated with sericin, a hydrophilic protein. Sericin is degummed during the silk purification process leaving the core fibers corresponding to silk. For tissue engineering applications, silk fibroin has shown to have better mechanical properties than other natural polymers, excellent biocompatibility and its degradation products are non-toxic (Bai et al. 2015).
On the other hand, sericin was considered to promote hypersensitivity reactions, however subsequent studies have shown that sericin as silk fibrin were immunologically inert in the culture of murine macrophage cells (Panilaitis et al. 2003). Sericin can be used as a biomaterial since some studies have demonstrated that there is no cytotoxicity for several cell lines when sericin is added to the culture media (Kunz et al. 2016).
Keratin is a fibrous protein, found in hair, wool, feathers, nails and horns of mammals, reptiles and birds. Keratin proteins can be classified in intermediate filament proteins and the matrix proteins. The characteristic secondary structure of intermediate filaments is a-helix, also known as α-keratins and are low in sulfur content. The matrix proteins are globular, have high sulfur content and are surrounding the intermediate filament proteins interacting through disulfide bonds (Magin et al. 2007). Keratin is characterized by the presence of sequences as RGD (Arg-Gly-Asp) and LDV (Leu-Asp-Val) found in several ECM proteins for cell adhesion. Thus, keratin has been proposed as an alternative to collagen for developing biomaterials for tissue regeneration (Srinivasan et al. 2010). Besides, several studies have shown that the addition of keratin and adjusting its concentration, improved the mechanical properties of biomaterials (Zhang et al. 2014; Wang et al. 2015).
Zein is a vegetable protein found in the endosperm of corn that has been explored for tissue engineering and drug delivery application due to its excellent biocompatibility (Dong et al. 2004; Zhang et al. 2016). The amino acid sequence is characterized by hydrophobic and neutral amino acids, and sole polar amino acids. Due to its composition, zein is a hydrophobic protein, which may contribute to controlling the material degradation for tissue engineering, and allowing longer and sustained release of drugs as carrier (Ali et al. 2014; Zhang et al. 2016).
Polysaccharides have been studied as promising biomaterials to design medical gels, scaffolds for tissue regeneration and controlled drug delivery. Polysaccharides are natural polymers that can be extracted from plant, alga, animal and microbial sources. This group of polymers is composed of monosaccharides linked by O-glycosidic linkages. They include chitosan, hyaluronic acid, alginate, cellulose and their derivates. All these materials show good biocompatibility, biodegradability and low toxicity, high hydrophilicity, excellent mucoadhesive properties and tailored chemistry (Guarino et al. 2017a; Ahmad et al. 2018).
Chitosan is derived from the N-deacetylation of chitin, found in the exoskeleton of crustaceans. Chitosan has been widely used for oral treatments due to its properties as bioadhesivity, biodegradability, biocompatibility and antimicrobial activity (Send 2010). Chitosan properties have led to developing different biomaterials as films, hydrogels and fibers for tissue engineering (Lan Levengood and Zhang 2015). Chitosan is a cationic polysaccharide because its primary amino groups are responsible for the sustained release of molecules, thus have been used widely for local drug delivery systems, and also for periodontal applications (Felt et al. 1998; Guarino et al. 2015).
Hyaluronic acid is a non-sulfated glycosaminoglycan, consisting of disaccharide units of D-glucuronic acid and n-acetyl-D-glucosamine. It is found in extracellular matrix of connective tissue, synovial fluid, vitreous humor, embryonic mesenchyme skin and other tissues in human body (Tiwari and Bahadur 2019). The high hydrophilicity of hyaluronic acid confers a particular rheological behavior and inherent pharmacological properties (Liu et al. 2017).
Lastly, alginate is a natural polysaccharide extracted from brown sea algae, composed of D-manuronic acid and L-guloronic acid. Alginate is a biocompatible, non-toxic, biodegradable and low-cost material (Kolambkar et al. 2011; Westhrin et al. 2015). Alginate-based materials have been used in the form of hydrogels, microspheres, microcapsules and fibers for tissue engineering and drug delivery systems. Alginate hydrogels or scaffolds can be prepared in the presence of cations such as Ca2+ at low concentrations via the ionic interaction between the cation and the carboxyl functional group of alginate (Guarino et al. 2015; Barron and He 2017). To improve the biological and mechanical properties of alginate materials, it has been used in combination with other synthetic or natural polymers and growth factors (Bonino et al. 2011; Lan et al. 2018). For encapsulation of cells, alginate is also a promising material for its ability to form microgels that can serve as a 3D reservoir (Yao et al. 2012).
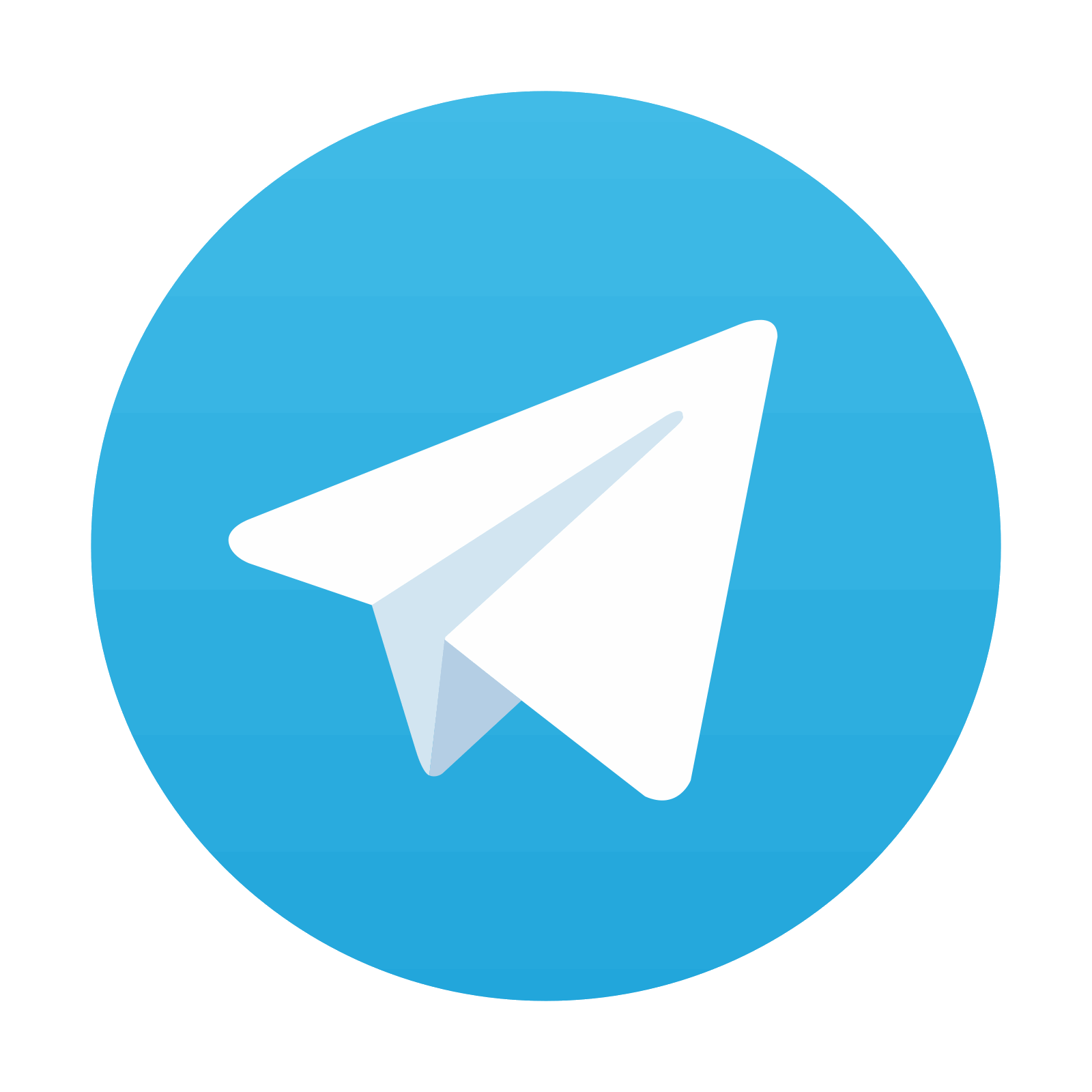
Stay updated, free dental videos. Join our Telegram channel

VIDEdental - Online dental courses
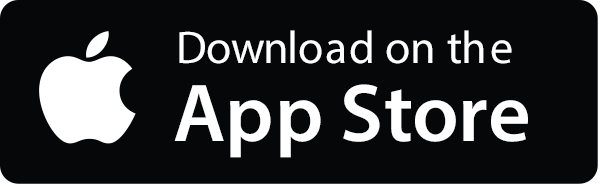
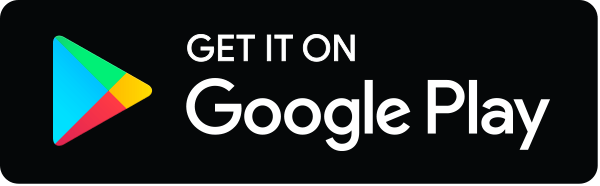