Abstract
Objective
The present work shows the optimization of a high-throughput bioluminescence assay to assess the metabolism of intact Streptococcus mutans biofilms and its utility as a screening method for nanofilled antibacterial dental materials.
Methods
The assay was optimized by monitoring changes in bioluminescence mediated by variation of the experimental parameters investigated (growth media and sucrose concentration, inoculum:D-Luciferin ratio, dilution factor, inoculum volume, luminescence wavelength, replicate and luciferase metabolic activity). Confocal microscopy was then used to demonstrate the impact of biofilm growth conditions on the 3-D distribution of extracellular polymeric substance (EPS) within Streptococcus mutans biofilms and its implications as confounding factors in high-throughput studies (HTS).
Results
Relative Luminescence Unit (RLU) values from the HTS optimization were analyzed by multivariate ANOVA ( α = 0.05) and coefficients of variation, whereas data from 3-D structural parameters and RLU values of biofilms grown on experimental antibacterial dental adhesive resins were analyzed using General Linear Models and Student–Newman–Keuls post hoc tests ( α = 0.05). Confocal microscopy demonstrated that biofilm growth conditions significantly influenced the quantity and distribution of EPS within the 3-D structures of the biofilms. An optimized HTS bioluminescence assay was developed and its applicability as a screening method in dentistry was demonstrated using nanofilled experimental antibacterial dental adhesive resins.
Significance
The present study is anticipated to positively impact the direction of future biofilm research in dentistry, because it offers fundamental information for the design of metabolic-based assays, increases the current levels of standardization and reproducibility while offering a tool to decrease intra-study variability.
1
Introduction
Bacterial viability is typically determined by counting the number of colony forming units (CFU) [ ] using methodologies that are costly, labor-intensive, technique-sensitive and time consuming (ranging from 16 to 72 h) [ , ]. Another disadvantage associated with the CFU method is the level of biofilm manipulation required to separate bacteria from EPS within biofilms by vortexing, sonication or matrix-dissolving enzymes [ ], which has been previously shown to impact cells’ viability and leads to inaccurate viable cell counts. This, combined with the additional limitations mentioned, make these methodologies unsuitable for longitudinal HTS studies [ ].
In this context, several assays capable of measuring bacterial viability based on metabolic reactions have been developed over the years [Crystal violet, Alamar Blue, 3-(4,5-dimethylthiazol-2-yl)-2,5-diphenyltetrazolium bromide (MTT) and 2,3-bis-(2-methoxy-4-nitro-5-sulfophenyl)-2h-tetrazolium-5-carboxanilide (XTT)]. However, despite many advantages reported in the literature [ ], these types of metabolic assays typically require the utilization of large inocula (greater than 5- or 6-log CFU) and calibration curves derived from planktonic bacteria to successfully quantify the viability of bacteria in biofilms [ ]. The latter requirement was demonstrated to introduce significant errors in the step of viability measurement due to marked differences in the metabolism of biofilms versus planktonic cells [ ].
Optical density (OD) can be measured automatically in a HTS manner using a multi-well plate reader to estimate bacterial load. However, this method is limited to a concentration threshold between 10 8 and 10 10 bacteria/mL, it is not capable of discerning between live/dead bacteria and suspended particulates and it is not technically feasible for intact biofilms. Other methods, such as confocal microscopy and flow cytometry, are less commonly used because they are time-consuming, require expensive equipment, trained personnel, and optical limitations restrict the efficiency of light collection to resolve subcellular structures [ ]. These factors combined have decreased enthusiasm for the utilization of these methods in HTS studies. Therefore, robust, precise and repeatable assays are critically needed to respond to recent expectations from the U.S. National Institutes of Health (NIH) for reproducible data [ ].
Fluorescent and bioluminescent assays are based on the emission and collection of photons that are typically generated when molecules, excited either by light irradiation (fluorescence) or oxidation reactions (bioluminescence), transition to their corresponding fundamental states [ ]. Due to their fundamental mechanisms, fluorescence assays are brighter, but display modest signal-to-noise ratios, which may adversely impact the sensitivity of these types of assays. In contrast, even though bioluminescence assays yield much lower light intensities, they also yield unrivaled signal-to-noise ratios, since photons are produced only from enzymatic reactions [ ]. Therefore, bioluminescent assays are typically considered excellent candidates to generate meaningful data with the necessary throughput, quality, accuracy and specificity needed to translate biomolecular reactions into measurable parameters. According to Thorne et al. [ ], these sophisticated assays are characterized by their ability to be translated into fully automated HTS systems capable of monitoring intricate biological processes.
Bioluminescent assays typically rely on the utilization of luciferase enzymes extracted from bioluminescence-producing organisms such as fireflies ( Photinus Pyralis , 62-kDa; Photuris pennsylvanica , 61-kDa), click beetle ( Pyrophorus plagiophthalamus , 60-kDa), sea pansy ( Renilla reniformis , 36-kDa) [ ] and copepod ( Gaussia princeps, 19-kDa) [ ]. The underlying mechanism by which these structurally diverse enzymes are capable of oxidizing luciferins into oxyluciferins, with the concurrent emission of photons [ ], has been previously described by the equation below by Shama and Malik [ ], that describes the bioluminescence metabolic reaction for beetle luciferases such as firefly and click beetle.
Luciferin+O2+ATP→luciferase,Mg2+Oxyluciferin+AMP+PPi+CO2+hν,
where ATP stands for adenosine triphosphate, AMP stands for adenosine monophosphate, PPi for pyrophosphate, and hν indicates emission of light.
Fan and Wood [ ] have stated that luciferase reporter assays may be configured for HTS while providing a simple and efficient method to measure cellular physiological events without sacrificing data quality, due to signal amplification by intracellular transduction and genetic transcription pathways. Esteban Florez et al. [ ] recently introduced and validated a non-destructive and real-time firefly assay based on a bacterial construct developed by Merritt et al. [ ] The bioluminescent assay was demonstrated to be capable of assessing the viability of intact Streptococcus mutans biofilms grown on resin composites, and displayed strong Spearman correlation values for non-disrupted ( r s = 0.766, p < 0.0001) and sonicated ( r s = 0.910, p < 0.0001) bacteria when compared to the CFU method [ ]. Although the newly developed and validated assay has numerous advantages, it was based on a single-tube luminometer, lacked the necessary high-throughput and automation capabilities required for the analysis of large data sets in longitudinal studies with repeated measures. With this in mind, we present the optimization of a real-time and non-disruptive firefly bioluminescence assay capable of assessing, in a high-throughput manner, the metabolism of non-disrupted cariogenic (caries-producing) biofilms. The optimized assay was then used to determine the impact of novel experimental dental adhesive resins containing either single-doped [nitrogen doping (N_TiO 2 )] or co-doped titanium dioxide nanoparticles [nitrogen and fluorine doping (NF_TiO 2 ) or nitrogen and silver doping (NAg_TiO 2 )] on the metabolism of intact S. mutans biofilms. The complete underlying mechanism of action of doped- or co-doped TiO 2 photocatalysis remains to be fully elucidated. However, the generation of reactive oxygen species (ROS) upon on-demand visible light irradiation (400–700 nm) along with lipid peroxidation are believed to be the major processes by which these nanoparticles express their antibacterial functionalities. Therefore, the rationale for the selection of these types of metaloxide nanoparticles was based on (i) the ability of nanoparticles to exert antibacterial properties when immobilized in current polymer compositions (in both dark and visible-light irradiated conditions), (ii) our ability to synthesize metaloxide nanoparticles using robust solvothermal reactions and (iii) a recent publication from our laboratory describing the antibacterial and bioactive properties of N_TiO 2 using viable colony counts (CFU/mL), live/dead staining, confocal microscopy and SEM/EDS, respectively [ ].
2
Materials and methods
2.1
Bacterial strain and in vitro growth of biofilms
A genetically modified and bioluminescent strain of Streptococcus mutans (JM10), a derivative of wild type UA159 constructed by Merritt et al. [ ] was used. Colonies of JM10 were grown on TH agar plates (Todd-Hewitt, BD Difco, USA) supplemented with 0.3% yeast extract (EMD Millipore, USA) and 800 μg/mL of Spectinomycin (MP Biomedicals, USA) under anaerobic conditions at 37 °C for 48 h for a total of two passages. A single colony was inoculated in 4.0 mL of THY broth with 32 μL of Spectinomycin (100 mg/mL), then incubated at 37 °C for 16 h (static cultures, anaerobic conditions). Planktonic cultures having optical densities (OD 600 ) equal to or higher than 0.900 (corresponding to 6.43 e +12 CFU/mL) were used for biofilm growth. Four dilutions of bacterial inoculum [1:50, 1:100, 1:250 and 1:500 (v/v)], three concentrations of THY biofilm growth media (0.35x, 0.65x, and 1x) and three concentrations of sucrose [0.1, 0.5, or 1.0% (w/v)] were the growth conditions tested. Each combination (inoculum + growth medium + sucrose) was then used to grow biofilms (n = 6/combination, total n = 10,368) in the wells of sterile white 24-well plates (catalog #6005816; Perkin-Elmer, USA) for either 24 or 48 h in anaerobic
conditions at 37 °C, as described in Table 1 .
Experimental groups | Media [] | Sucrose [%] | Dilution | Inoculum volume (μL) | Inoculum: D-Luciferin ratio (v/v) | Growth (h) | Luminescence wavelength (nm) |
---|---|---|---|---|---|---|---|
G1 (n = 6/growth condition, total = 3456) | 0.35x | 0.1 | 1:500 | 300 | 5:1 | 24 | 530 |
0.65x | 1:250 | 500 | 2:1 | 48 | 590 | ||
1x | 1:100 | 750 | |||||
1:50 | 1000 | ||||||
G2 (n = 6/growth condition, total = 3456) | 0.35x | 0.5 | 1:500 | 300 | 5:1 | 24 | 530 |
0.65x | 1:250 | 500 | 2:1 | 48 | 590 | ||
1x | 1:100 | 750 | |||||
1:50 | 1000 | ||||||
G3 (n = 6/growth condition, total = 3456) | 0.35x | 1.0 | 1:500 | 300 | 5:1 | 24 | 530 |
0.65x | 1:250 | 500 | 2:1 | 48 | 590 | ||
1x | 1:100 | 750 | |||||
1:50 | 1000 |
2.2
Bioluminescence assay
After the growth period of either 24 or 48 h, biofilms were removed from the incubator, the media was aspirated and the biofilms were then washed twice with phosphate buffered saline (PBS, pH 7.4) for the removal of non-adherent cells following a modified protocol previously published by our laboratory. [ ] Briefly, aliquots (1.0 mL/well) of room-temperature PBS were carefully dispensed into the wells containing biofilms using a serological pipette. Biofilms in PBS were then washed for 15 s at 150 revolutions per minute (RPM) using an orbital shaker (KS 260, IKA-Werke GmbH & Co. KG, Germany). After that, 1x THY + 1% glucose culture medium (recharge medium) was added to biofilms in the same volume as the original inoculum volume (either 300, 500, 750 or 1000 μL). Recharged biofilms were then incubated (37 °C, 1 h) in preparation for bioluminescence testing. D-Luciferin aqueous solution (100 mM) suspended in 0.1 M citrate buffer (pH 6.0) was added by a computer-controlled system in a Synergy HT Multi-mode microplate reader (Biotek, USA) to the wells containing both the biofilms and recharge medium in either 5:1 or 2:1 ratio [(v/v) of inoculum: D-Luciferin]. The assessment of luciferase metabolic activity in non-disrupted S. mutans biofilms was evaluated in 2-min increments (6 min total) after the addition of D-Luciferin substrate at 530 and 590 nm. These two wavelengths were chosen to demonstrate the relationship between wavelength and RLU values.
2.3
Nanoparticles’ synthesis
Nitrogen-doped TiO 2 nanoparticles (N_TiO 2 ) were synthesized via a 2-step process wherein the first step was the solvothermal synthesis of pure TiO 2 in a manner similar to Dinh et al. [ ], and the second step was doping of the TiO 2 in a manner similar to Huo et al. [ ] In the first step, a solution comprised of 1.7 g Ti(IV)-butoxide (Aldrich, 97%), 4.6 g ethanol (Decon Labs, 200 proof), 6.8 g oleylamine (Aldrich, 70%), and 7.1 g oleic acid (Aldrich, 90%) was prepared, then mixed with 20 mL of 4% H 2 O in ethanol (18-MΩ Milli-Q; Decon Labs). This solution was then split into two aliquots (≈20 mL/aliquot). Each aliquot was then placed into a high-pressure reaction vessel (Paar Series 5000 Multiple Reactor System) and reacted at 180 °C for 24 h. External magnetic field and Teflon-coated stir bars were used to stir the vessels. The reaction vessels were Teflon-lined. After reaching room temperature, the solutions were decanted and washed 3 times with anhydrous ethanol to remove extraneous surfactants. The pure TiO 2 nanoparticles were readily dispersible into 20–30 mL ethanol, but did not form clear solutions. In the doping step, a portion of the TiO 2 nanoparticles in ethanol were then reacted with equal volume of triethylamine (Aldrich, 99.5%) at 140 °C for 12 h in the high-pressure reaction vessel. Upon cooling, the now-doped N_TiO 2 particles were rinsed 3 times with anhydrous ethanol. The final N_TiO 2 nanoparticle solution was in ethanol, and the particles’ concentration was determined gravimetrically (≈35 mg/mL).
2.3.1
Synthesis of Co-doped nanoparticles
Additionally, two other types of TiO 2 nanoparticles were synthesized and doped using variations of the first and second steps in Section 2.4 above. Variation 1: Nitrogen and Silver co-doping (NAg_TiO 2 ) was obtained by a single reaction based on step 1 above, with the inclusion of 5% Ag (based on Ti content) and 5% N, using silver acetlyacetonate and tetramethyl ammonium hydroxide as the dopant sources. Variation 2: Nitrogen and Fluorine co-doping (NF_TiO 2 ) was obtained by a single reaction based on step 1 above, with the inclusion of 5% F (based on Ti content) and 5% N, using ammonium fluoride as the dopant source. In both variations, the TiO 2 nanoparticles formed as usual with the dopants in place.
2.4
Specimen fabrication
Disk shaped specimens (n = 18/group; diameter = 6.00 mm, thickness = 0.50 mm) of unaltered OptiBond Solo Plus (Kerr Corp., OPTB), Adper Scotchbond (3 M ESPE, SCTB), Clearfil SE Protect (Kuraray Co., CLRF) and experimental dental adhesive resins [OPTB + 30% (v/v) of either N_TiO 2 , NAg_TiO 2 or NF_TiO 2 (Oak Ridge National Laboratory, USA)] were fabricated, light cured (160 s/specimen, UltraLume 5, Ultradent Products, Inc., USA) and UV-sterilized (254 nm, 800,000 μJ/cm 2 , UVP Crosslinker, model CL-1000, UVP, USA). Specimens were then transferred to 24-well plates containing sterile ultrapure water (1000 μL/well) and were incubated at 37 °C for 24 h for the extraction of unreacted monomers.
2.5
Optimized HTS viability assessment of S. mutans biofilms on experimental antibacterial adhesive resins
Biofilms of S. mutans (JM10) were grown on the surfaces of specimens (n = 18/group) fabricated from unaltered (OPTB, SCTB, CLRF) and experimental dental adhesive resins containing 30% (v/v) of either N_TiO 2 , NF_TiO 2 or NAg_TiO 2 to assess the impact of antibacterial materials on the viability of intact biofilms. Planktonic cultures of S. mutans (JM10) were grown in THY culture medium at 37 °C for 16 h. Planktonic cultures having optical density (OD 600 ) levels equal to or higher than 0.900 (corresponding to 6.43 e +12 CFU/mL) were used as inoculum to grow the biofilms. Optimal biofilm growth parameters identified during the HTS optimization [1:50 dilution, 0.65x THY + 1% (w/v) sucrose, 1000 μL] were then used to grow the biofilms. Aliquots (1.0 mL) of inoculated biofilm growth media were dispensed into the wells of sterile 24-well microtiter plates (Falcon, Corning, USA) containing sterile specimens. Biofilms were grown for either 24 or 48 h (static cultures, anaerobic conditions, 37 °C) with or without continuous light irradiation provided by a prototype LED device (410 ± 10 nm, 24-h irradiation = 310.07 J/cm 2 , 48-h irradiation = 620.14 J/cm 2 ). An additional set of specimens fabricated with OPTB was treated with 2% chlorhexidine gluconate (CHX) for 2 min and served as the negative control group. After the growth period, biofilms were replenished with 1.0 mL of fresh 1x THY + 1% (w/v) glucose recharge medium and were incubated at 37 °C for 1 h. Replenished biofilms were transferred into the wells of sterile white 24-well plates containing 1.0 mL of fresh 0.65x THY + 1% (w/v) sucrose medium. D – Luciferin aqueous solution (100 mM) suspended in 0.1 M citrate buffer (pH 6.0) was added by a computer-controlled system in a Synergy HT Multi-mode microplate reader (Biotek, USA) to the wells containing both the specimens with biofilms and recharge medium in 2:1 ratio [(v/v) of inoculum: D Luciferin]. Luciferase metabolic activity in non-disrupted S. mutans biofilms was evaluated at 590 nm in 2-min increments (6 min total) after the addition of D-Luciferin substrate in terms of Relative Luminescence Units (RLUs).
2.6
Staining and confocal microscopy
A confocal microscopy assay was necessary to demonstrate the impact of biofilm growth conditions on the distribution of biofilm components nucleic acid, proteins and extracellular polymeric substances. To this end, an additional set of biofilms (n = 4/condition) were grown on sterile glass slides for 24 h using specific inoculum dilutions (1:50, 1:100, 1:250 and 1:500) and THY growth medium concentrations (0.35x, 0.65x and 1x) supplemented with 0.1% sucrose. Biofilms were then washed three times with PBS (pH 7.4, 25 °C, 15 s/wash) to remove non-adherent cells in preparation for concurrent staining with Alexa Fluor® 647 conjugate of Concanavalin A (Invitrogen, USA; 250 μg/mL), Syto 9 (Molecular Probes, USA; 10 μM), and Sypro Red (Invitrogen, USA; 10x), which are specific for the EPS, nucleic acid, and protein components of the biofilms, respectively, as described by Khajotia et al. [ ] Biofilms were kept hydrated in sterile ultra-pure water and protected from light until confocal microscopy.
Biofilms were then imaged in sterile ultrapure water using a TCS-SP2 MP confocal laser scanning microscope (CLSM, Leica Microsystems, Inc., USA) with Ar (488 nm) and He/Ne (543 and 633 nm) lasers for excitation of the fluorescent stains. A 63x water immersion microscope objective lens was used. Serial optical sections were recorded from the top of glass slides to the top of the biofilms at 0.6 μm intervals in the z-direction. Four specimens were tested per group and three locations were scanned on each specimen, resulting in 12 CLSM images per group. Two 3-dimensional biofilm structural parameters [biovolume (BV) and mean biofilm thickness (MTBF)] were calculated for each biofilm component (fluorescent stain) using ISA3D software, as described by Khajotia et al. [ ]. Representative 3-D images of the biofilms were generated using Volocity software (PerkinElmer, USA) to help with visualization of the distribution of the nucleic acid, proteins and EPS components of the biofilms.
2.7
Statistical analyses
RLU values obtained during the optimization of the HTS bioluminescence assay for each combination of experimental variables investigated shown in Table 1 (inoculum dilution [1:50, 1:100, 1:250 and 1:500], THY [0.35x, 0.65x, and 1x] and sucrose concentration [0.1, 0.5, or 1.0%]) were analyzed using multivariate ANOVA and coefficients of variation (CV). Data for each of the 3-D structural parameters (BV and MTBF) were analyzed using General Linear Models (GLM) and post hoc Student Newman Keuls tests (SNK; α = 0.05). RLU values indicating the viability of intact biofilms of S. mutans grown against the surfaces of both unaltered and experimental dental adhesive resins were also compared using GLM and SNK post hoc tests ( α = 0.05). All statistical analyses were performed with the Statistical Analysis System (SAS software, version 9.2; SAS Institute, USA).
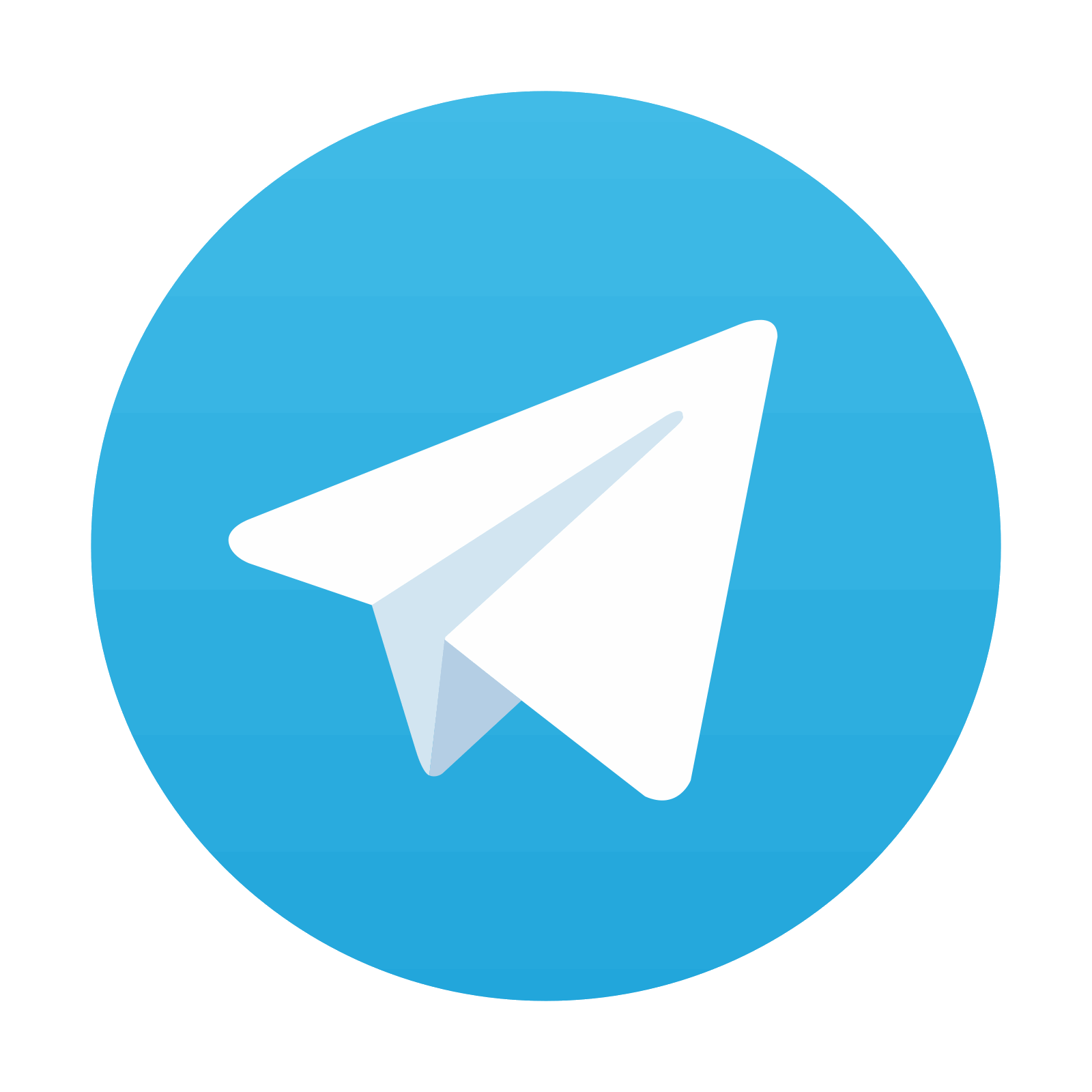
Stay updated, free dental videos. Join our Telegram channel

VIDEdental - Online dental courses
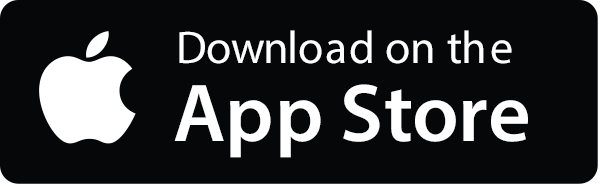
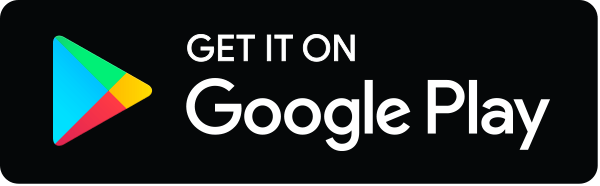