Abstract
Objectives
To evaluate the effects of chlorhexidine (CHX) addition in different concentrations into simplified etch-and-rinse adhesives on the ultimate tensile strength (UTS), water sorption (WS), solubility (SO) and the rate of CHX release over time.
Methods
We added CHX diacetate to Ambar [AM] (FGM) and XP Bond [XP] (Dentsply) in concentrations of 0, 0.01, 0.05, 0.1 and 0.2 wt%. For UTS ( n = 10 for each group), adhesive specimens were constructed in an hourglass shape metallic matrix with cross-sectional area of 0.8 mm 2 . Half of specimens were tested after 24 h and the other half after 28 days of water storage in tension of 0.5 mm/min. For WS and SO ( n = 10 for each group), adhesive discs (5.8 mm × 1.0 mm) were prepared into a mold. After desiccation, we weighed and stored the cured adhesive specimens in distilled water for evaluation of the WS, SO and the cumulative release of CHX over a 28-day period. For CHX release ( n = 10 for each group), spectrophotometric measurements of storage solution were performed to examine the release kinetics of CHX. We subjected data from each test to ANOVA and Tukey’ test ( α = 0.05).
Results
XP Bond adhesive showed significantly more WS and SO and lower UTS than Ambar. In general, the addition of CHX did not alter WS, SO and UTS of the adhesives. XP showed a higher CHX release than AM ( p < 0.05) in all concentrations and the final amount of CHX release was directly proportional to the initial CHX concentration added to the adhesives. After 28 days of water storage, approximately 20% of CHX was released from XP and 8.0–12.0% from AM.
Conclusions
Addition of CHX to commercial adhesive is a feasible method to provide a controlled release of CHX over time without jeopardizing WS, SO and UTS of the adhesives.
Significance
Manufacturers should consider adding CHX to commercial adhesives to provide a controlled release of CHX over time.
1
Introduction
Biodegradation of resin-bonded interfaces has been a focus of recent studies. We do not entirely yet understand why the hybrid layer degrades . However, it seems that biodegradation begins with extraction of the resins that had infiltrated the dentin matrix via water-filled, nanometer-sized voids, followed by the host-derived enzymatic attack of exposed collagen fibrils .
Pashley et al. were the first to demonstrate the role of host-derived enzymes on the depletion of collagen fibrils by assaying the collagenolytic activity of human mineralized dentin powder, exerted by host-derived dentin metalloproteinases (MMPs) , which others had theorized were expressed in the dentin–pulp complex .
Since then, several studies have focused their investigations on how MMP inhibitors may prolong the resin dentin bonds’ durability. The use of 2% chlorhexidine digluconate (CHX digluconate) for 60 s as a preliminary dentin treatment after acid-etching has reduced interfacial aging over time, by inhibiting the activation of endogenous MMPs . This procedure, however, adds another step to the bonding protocol, despite the clinician’s need for simplification.
More simplified protocols, using low concentrates of CHX, reduced application times and CHX incorporation into the acid etch gel has successfully preserved the stability of the resin-dentin bonds after 6–12 months . This medium-term efficacy of CHX has been attributed to its substantivity , since large amounts of CHX remain bound to partially and completely demineralized dentin incubated in saline solution for at least 8 weeks .
On the other hand, over longer periods of time such as 18–24 months, the same interfaces treated with CHX show slight and progressive signals of hybrid layer degradation . A CHX reservoir is not produced right after acid-etching; thus CHX may be rapidly leached out from the hybrid layer. We hypothesize that any approach that could provide this controlled release of CHX around collagen fibrils could add even more to the life expectancy of resin–dentin adhesive restorations by slightly transferring CHX to adjacent collagen .
The inclusion of CHX into primers and/or adhesives could produce a reservoir for controlled release. Earlier studies that added CHX in primers and/or adhesives and investigated the durability of the resin–dentin bonds have shown controversial conclusions . This alternative seems to depend on the concentration of CHX added to the adhesive formulation as well as the chemical compositions of the adhesives to which CHX was included. This deserves further testing.
We do not yet know the kinetics of such CHX release or the influence of CHX concentration on such property. Only one study has profiled CHX release from adhesives, and it used non-solvated experimental adhesives with varied hydrophilias . Therefore, we designed this in vitro study to investigate the ultimate tensile strength (UTS), water sorption (WS), solubility (SO) and CHX release kinetics from two commercial simplified etch-and-rinse adhesive systems after adding different amounts of CHX diacetate.
2
Materials and methods
2.1
Formulation of the experimental adhesives
We formulated experimental resins using the simplified etch-and-rinse adhesive systems XP Bond [XP] (Dentsply, York, PA, USA) and Ambar [AM] (FGM Prod. Odont. Ltda, Joinville, SC, Brazil). Table 1 depicts their detailed composition, mode of application and batch number. We added five different concentrations of CHX diacetate to the resins (99.9% pure, Sigma Chemical, St. Louis, MO, USA) (wt%): 0% (control, commercial material), 0.01%, 0.05%, 0.1% and 0.2%. The CHX was added to the adhesive and mechanically mixed by a motorized mixer (stirring).
Adhesive systems | Composition | Bach number |
---|---|---|
XP Bond (XP – Denstsply) |
TCB-resin, terc -butyl alcohol, PENTA, PPD, UDMA, TEGDMA, HEMA, modified carboxylic acid, nanofiller and dimethacrylate | 0804002271 |
Ambar (AM – FGM Prod. Odontológicos) |
Methacrylate monomers (UDMA and MDP), photoinitiatiors, co-initiators, stabilizers, inert silica nanoparticles and ethanol | 200910 |
2.2
Ultimate tensile strength (UTS)
A metallic matrix (10 mm long, 2 mm wide, and 1 mm deep with an hourglass shape) was employed for the construction of the bonding resin specimens. After isolating the matrix with a petroleum jelly, we dropped each experimental adhesive into the mold with air-dried oil and water-free compressed air (40 s) to allow for solvent evaporation. Then we placed a plastic matrix strip on the adhesive and light-cured the surface for 60 s with an LED light source operated at 1200 mW/cm 2 (Radii-cal, SDI, Bayswater, Victoria, Australia). Such prolonged exposure was required to keep XP Bond from getting a rubbery and sticky appearance as it did in shorter tests (not shown data). We tested half of the specimens 24 h after preparation and stored the other half in water for 28 days at 37 °C. We attached each to a modified device with cyanoacrylate resin and subjected to a tensile force at 0.5 mm/min. Ten specimens reflected each experimental condition were tested.
2.3
Water sorption (WS) and solubility (SO)
Ten resin discs of each material were produced in a brass mold (5.8 mm diameter, 1.0 mm thick). We directly dispensed the liquid adhesive to completely fill the mold, carefully removing all visible air bubbles trapped in the adhesives were carefully removed. An air stream evaporated the solvent for 40 s at a distance of 10 cm.
Under a glass cover slip, the adhesive was light-cured for 40 s by an LED light source at 600 mW/cm 2 (Radii-cal, SDI). With the adopted energy density (4.8 J/cm 2 ) specimens allowed removal from the brass mold without undergoing permanent deformation.
The ISO specification 4049 helped determine WS and SO , except for specimens’ dimensions . Immediately after polymerization, we placed the specimens in desiccators, transferred them to a pre-conditioning oven at 37 °C, and left them undisturbed for 5 days. After that, we weighed them at 24-h intervals until day-to-day variation of mass dropped below 0.2 mg. A digital caliper measured specimens’ thickness and diameter, and rounded to the nearest 0.01 mm, these measurements helped calculate the volume ( V ) of each specimen (in mm 3 ).
We then placed each in a sealed Eppendorf containing 10 mL of distilled water (pH 7.2) at 37 °C. After fixed time intervals of 1, 2, 3, 4, 5, 6, 7, 14 and 28 days of storage, we removed the Eppendorfs from the oven and left them at room temperature for 30 min. We then washed the specimens in running water, gently wiped them with a soft absorbent paper, weighed them in an analytical balance ( m 2) and returned them to the vials with 10 mL fresh water. After the 28-day storage time, desiccators containing fresh silica gel in an oven dried the specimens at 37 °C, where they remained undisturbed for 10 days or until day-to-day weighing variations dropped below 0.2 mg, yielding constant mass ( m 3). We then measured each day-to-day mass result against the pre-Eppendorf mass ( m 1) to calculate the change in mass after each fixed time interval, during the 28 days of storage in water. We plotted changes in mass against the storage time to obtain the kinetics of WS during the entire period of water storage.
WS and SO over the 28 days of water storage were calculated using the following formulae: WS = ( m 2 − m 3)/ V and SL = ( m 1 − m 3)/ V .
2.4
Release behavior of CHX from adhesives
We used a series of standard solutions containing 1, 5, 10, 15, 20, 25, 30, 35, 40, 45 and 50 μg/mL of CHX diacetate to obtain an analytical curve with a linear regression between absorbance values and CHX concentrations, using a Genesys 10S UV-Vis Spectrophotometer (Thermo Scientific, Madison, WI, USA). We confirmed the maximum absorbance of CHX at 260 nm.
We produced ten new resin discs of each material as described for WS and SO tests, individually storing them in deionized water. At appropriate time intervals (1, 3 and 12 h and 1, 2, 3, 4, 5, 6, 7, 10, 12, 14, 21, 28 and 180 days), we obtained absorbance values of these storage solutions at 260 nm and converted them to quantities of CHX released based on the linear analytical curve. Thus, we subtracted the UV absorbance value at 260 nm of controls (0% CHX discs) from values produced from CHX-containing discs, considering the concomitant monomer release. We represented the cumulative release for the 28-day period as the percentage of CHX released and as the mass (mg) of CHX release per gram of the adhesive sample .
MicroMath Scientist™ 2.01 software (Salt Lake City, UT, USA) used mathematical models to build cumulative profiles of CHX released from resin discs and evaluate CHX release behavior. We tested data to fit first-order, biexponential, zero-order, Weibull, and monolag equations ( Table 2 ). We chose the best fit considering the correlation coefficient ( r ), the model selection criteria (MSC), and graphical adjustment.
Model | Equation |
---|---|
First-order | % D = 100(1 − e − kt ) |
Biexponential | % D = 100[1 − ( Ae − αt + Be − βt )] |
Zero-order | % D = kt |
Weibull | % D = 100[1 − e −( t / TD ) b ] |
Monolag | % D = 100[1 − e − k ( t − x ) ] |
To get some insight into the CHX release mechanism, we also used a very simple and semi-empirical equation to describe drug release from polymeric systems, the power law (Korsmeyer–Peppas model): ft = a · t n . In this equation, ft is the dissolved fraction of CHX at time t , n is the release exponent, indicative of the mechanism of the substance release and a is the constant incorporating structural and geometric features of the resin disk. After identification of the best model that described the release of CHX from adhesive systems, we calculated the time required to allow a 50% release of CHX from each material, assuming this equation remained as the dominating release mechanism over time, using MathWorks Matlab™ R2012a software (Natick, MA, USA) .
2.5
Statistical analysis
The means and standard deviations of UTS (MPa), WS (%), SO (%) and CHX release (% and mg/g) were calculated for all experimental conditions. Data from UTS were analyzed by three-way ANOVA using the adhesive, CHX concentration, and storage period as the main factors. For WS, SO, and CHX release the 28-day data were analyzed by two-way ANOVA using the adhesive and CHX concentration as the main factors. We also used Tukey’s test for multiple post hoc comparisons. We preset statistical significance at α = 0.05 and evaluated the correlations between WS and SO and the cumulative release of CHX with linear regression analysis ( α = 0.05).
2
Materials and methods
2.1
Formulation of the experimental adhesives
We formulated experimental resins using the simplified etch-and-rinse adhesive systems XP Bond [XP] (Dentsply, York, PA, USA) and Ambar [AM] (FGM Prod. Odont. Ltda, Joinville, SC, Brazil). Table 1 depicts their detailed composition, mode of application and batch number. We added five different concentrations of CHX diacetate to the resins (99.9% pure, Sigma Chemical, St. Louis, MO, USA) (wt%): 0% (control, commercial material), 0.01%, 0.05%, 0.1% and 0.2%. The CHX was added to the adhesive and mechanically mixed by a motorized mixer (stirring).
Adhesive systems | Composition | Bach number |
---|---|---|
XP Bond (XP – Denstsply) |
TCB-resin, terc -butyl alcohol, PENTA, PPD, UDMA, TEGDMA, HEMA, modified carboxylic acid, nanofiller and dimethacrylate | 0804002271 |
Ambar (AM – FGM Prod. Odontológicos) |
Methacrylate monomers (UDMA and MDP), photoinitiatiors, co-initiators, stabilizers, inert silica nanoparticles and ethanol | 200910 |
2.2
Ultimate tensile strength (UTS)
A metallic matrix (10 mm long, 2 mm wide, and 1 mm deep with an hourglass shape) was employed for the construction of the bonding resin specimens. After isolating the matrix with a petroleum jelly, we dropped each experimental adhesive into the mold with air-dried oil and water-free compressed air (40 s) to allow for solvent evaporation. Then we placed a plastic matrix strip on the adhesive and light-cured the surface for 60 s with an LED light source operated at 1200 mW/cm 2 (Radii-cal, SDI, Bayswater, Victoria, Australia). Such prolonged exposure was required to keep XP Bond from getting a rubbery and sticky appearance as it did in shorter tests (not shown data). We tested half of the specimens 24 h after preparation and stored the other half in water for 28 days at 37 °C. We attached each to a modified device with cyanoacrylate resin and subjected to a tensile force at 0.5 mm/min. Ten specimens reflected each experimental condition were tested.
2.3
Water sorption (WS) and solubility (SO)
Ten resin discs of each material were produced in a brass mold (5.8 mm diameter, 1.0 mm thick). We directly dispensed the liquid adhesive to completely fill the mold, carefully removing all visible air bubbles trapped in the adhesives were carefully removed. An air stream evaporated the solvent for 40 s at a distance of 10 cm.
Under a glass cover slip, the adhesive was light-cured for 40 s by an LED light source at 600 mW/cm 2 (Radii-cal, SDI). With the adopted energy density (4.8 J/cm 2 ) specimens allowed removal from the brass mold without undergoing permanent deformation.
The ISO specification 4049 helped determine WS and SO , except for specimens’ dimensions . Immediately after polymerization, we placed the specimens in desiccators, transferred them to a pre-conditioning oven at 37 °C, and left them undisturbed for 5 days. After that, we weighed them at 24-h intervals until day-to-day variation of mass dropped below 0.2 mg. A digital caliper measured specimens’ thickness and diameter, and rounded to the nearest 0.01 mm, these measurements helped calculate the volume ( V ) of each specimen (in mm 3 ).
We then placed each in a sealed Eppendorf containing 10 mL of distilled water (pH 7.2) at 37 °C. After fixed time intervals of 1, 2, 3, 4, 5, 6, 7, 14 and 28 days of storage, we removed the Eppendorfs from the oven and left them at room temperature for 30 min. We then washed the specimens in running water, gently wiped them with a soft absorbent paper, weighed them in an analytical balance ( m 2) and returned them to the vials with 10 mL fresh water. After the 28-day storage time, desiccators containing fresh silica gel in an oven dried the specimens at 37 °C, where they remained undisturbed for 10 days or until day-to-day weighing variations dropped below 0.2 mg, yielding constant mass ( m 3). We then measured each day-to-day mass result against the pre-Eppendorf mass ( m 1) to calculate the change in mass after each fixed time interval, during the 28 days of storage in water. We plotted changes in mass against the storage time to obtain the kinetics of WS during the entire period of water storage.
WS and SO over the 28 days of water storage were calculated using the following formulae: WS = ( m 2 − m 3)/ V and SL = ( m 1 − m 3)/ V .
2.4
Release behavior of CHX from adhesives
We used a series of standard solutions containing 1, 5, 10, 15, 20, 25, 30, 35, 40, 45 and 50 μg/mL of CHX diacetate to obtain an analytical curve with a linear regression between absorbance values and CHX concentrations, using a Genesys 10S UV-Vis Spectrophotometer (Thermo Scientific, Madison, WI, USA). We confirmed the maximum absorbance of CHX at 260 nm.
We produced ten new resin discs of each material as described for WS and SO tests, individually storing them in deionized water. At appropriate time intervals (1, 3 and 12 h and 1, 2, 3, 4, 5, 6, 7, 10, 12, 14, 21, 28 and 180 days), we obtained absorbance values of these storage solutions at 260 nm and converted them to quantities of CHX released based on the linear analytical curve. Thus, we subtracted the UV absorbance value at 260 nm of controls (0% CHX discs) from values produced from CHX-containing discs, considering the concomitant monomer release. We represented the cumulative release for the 28-day period as the percentage of CHX released and as the mass (mg) of CHX release per gram of the adhesive sample .
MicroMath Scientist™ 2.01 software (Salt Lake City, UT, USA) used mathematical models to build cumulative profiles of CHX released from resin discs and evaluate CHX release behavior. We tested data to fit first-order, biexponential, zero-order, Weibull, and monolag equations ( Table 2 ). We chose the best fit considering the correlation coefficient ( r ), the model selection criteria (MSC), and graphical adjustment.
Model | Equation |
---|---|
First-order | % D = 100(1 − e − kt ) |
Biexponential | % D = 100[1 − ( Ae − αt + Be − βt )] |
Zero-order | % D = kt |
Weibull | % D = 100[1 − e −( t / TD ) b ] |
Monolag | % D = 100[1 − e − k ( t − x ) ] |
To get some insight into the CHX release mechanism, we also used a very simple and semi-empirical equation to describe drug release from polymeric systems, the power law (Korsmeyer–Peppas model): ft = a · t n . In this equation, ft is the dissolved fraction of CHX at time t , n is the release exponent, indicative of the mechanism of the substance release and a is the constant incorporating structural and geometric features of the resin disk. After identification of the best model that described the release of CHX from adhesive systems, we calculated the time required to allow a 50% release of CHX from each material, assuming this equation remained as the dominating release mechanism over time, using MathWorks Matlab™ R2012a software (Natick, MA, USA) .
2.5
Statistical analysis
The means and standard deviations of UTS (MPa), WS (%), SO (%) and CHX release (% and mg/g) were calculated for all experimental conditions. Data from UTS were analyzed by three-way ANOVA using the adhesive, CHX concentration, and storage period as the main factors. For WS, SO, and CHX release the 28-day data were analyzed by two-way ANOVA using the adhesive and CHX concentration as the main factors. We also used Tukey’s test for multiple post hoc comparisons. We preset statistical significance at α = 0.05 and evaluated the correlations between WS and SO and the cumulative release of CHX with linear regression analysis ( α = 0.05).
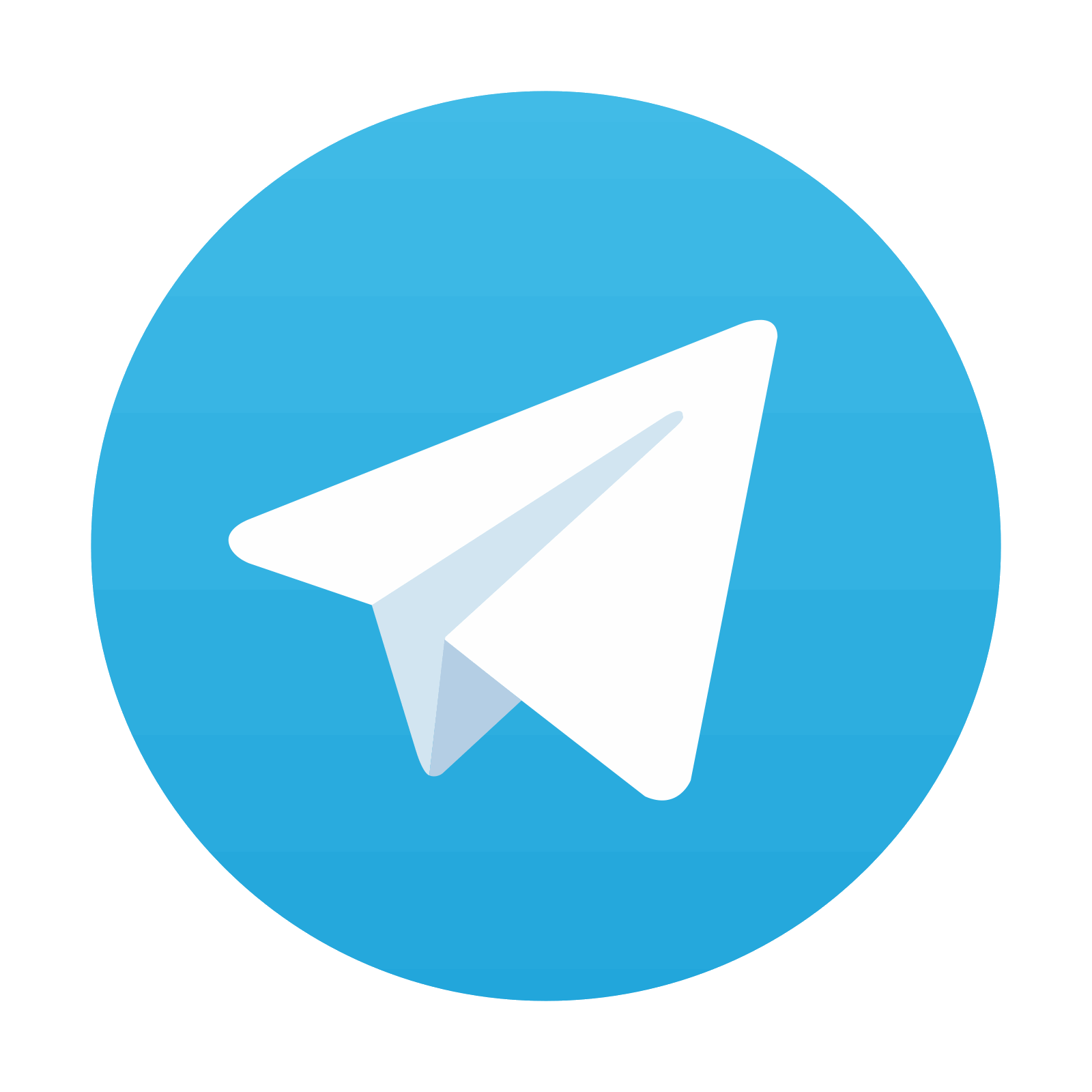
Stay updated, free dental videos. Join our Telegram channel

VIDEdental - Online dental courses
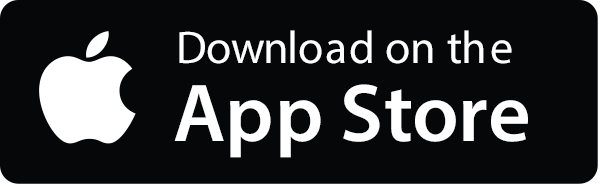
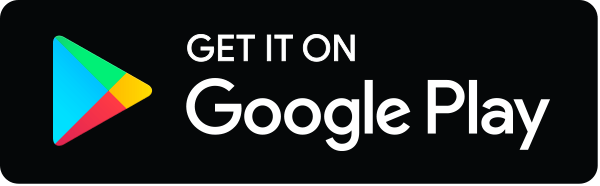