Abstract
Objective
To investigate, by means of FE analysis, the effect of surface roughness treatments on the distribution of stresses at the bone–implant interface in immediately loaded mandibular implants.
Materials and methods
An accurate, high resolution, digital replica model of bone structure (cortical and trabecular components) supporting an implant was created using CT scan data and image processing software (Mimics 13.1; Materialize, Leuven, Belgium). An anatomically accurate 3D model of a mandibular-implant complex was created using a professional 3D-CAD modeller (SolidWorks, DassaultSystèmes Solid Works Corp; 2011). Finite element models were created with one of the four roughness treatments on the implant fixture surface. Of these, three were surface treated to create a uniform coating determined by the coefficient of friction ( μ ); these were either (1) plasma sprayed or porous-beaded ( μ = 1.0), (2) sandblasted ( μ = 0.68) or (3) polished ( μ = 0.4). The fourth implant had a novel two-part surface roughness consisting of a coronal polished component ( μ = 0.4) interfacing with the cortical bone, and a body plasma treated surface component ( μ = 1) interfacing with the trabecular bone. Finite element stress analysis was carried out under vertical and lateral forces.
Results
This investigation showed that the type of surface treatment on the implant fixture affects the stress at the bone–implant interface of an immediately loaded implant complex. Von Mises stress data showed that the two-part surface treatment created the better stress distribution at the implant–bone interface.
Significance
The results from this FE computational analysis suggest that the proposed two-part surface treatment for IL implants creates lower stresses than single uniform treatments at the bone–implant interface, which might decrease peri-implant bone loss. Future investigations should focus on mechanical and clinical validation of these FE results.
1
Introduction
Immediate loading (IL) of dental implants has generated significant interest as a strategy for tooth replacement . In 1980, the IL concept that was first introduced by Ledermann et al., refers to the early (less than two days post-surgery) placement of the abutment and restoration in occlusal contact . The rationale behind the IL concept is to confer an immediate restoration of comfort, esthetics and psychological well-being to the patient with a reduced healing time . It has been demonstrated that the success of the IL is comparable to that of delayed loading (DL) implants after osseo-integration . Success rates for IL are encouraging with a reported failure rate of 11.2% in a retrospective study by Glauser et al. . A follow-up study performed by Calandriello et al. in 2003 reports a survival rate of 100% with no biomechanical problems detected for fifty patients that had received IL implants . Furthermore, a comparative evaluation undertaken by Bischof et al. in 2004 showed no difference between the stability of immediate and delayed loading implants with survival rates of 98.4% and 97.7%, respectively . Additionally, a higher degree of bone–implant contact and osseo-integration was reported for IL implants based on the histological analysis . High levels of metabolic activity has also been observed in IL implants .
The success of both IL and DL implants is dependent upon a range of factors; the host (systemic and local parameters) , the surgical procedure (site selection and operator skill) , and the nature of the implant itself (design, material and manufacturing process) . With regards to the design characteristics, the overall geometry affects the nature of stress distribution from the implant into the bone, which in turn affects its overall success or failure . Several numerical and retrospective follow-up studies have evaluated the effects of the geometrical factors on implant survival, including the length and width , macrostructure , thread types , and surface roughness . Whilst it is well recognized that primary stability of a dental implant relies on the macrostructure of the implant , secondary stability that results as a consequence of osseo-integration, is highly dependent on the surface microstructure . IL implants require a high level of primary stability in the early days post-insertion as these are loaded before secondary stability is achieved, and hence, early stress distribution and the effect of this on primary stability have been highlighted . In a numerical study carried out by Huang et al. in 2010, the effects of various geometries and also surface roughness of the immediately loaded implants have been investigated. Their results are validated by prototype experiments indicated that the magnitude of stresses in IL cases are strongly dependent upon the types of implants. Surface roughening is a strategy that is advocated for promoting an increased cellular interaction at the bone–implant interface, thus improving osseo-integration . The techniques for achieving this, such as plasma-spraying, porous beading, acid etching, sandblasting and polishing methods, are well documented . However, the nature (location and magnitude) of stress levels at the roughened coronal aspect of IL implants is poorly understood. Equally, little is known about the effect of these early stress levels on the ability to achieve secondary stability by means of osseo-integration.
Stress analysis can be effectively studied by sophisticated Finite Element Analysis (FEA) based on computerized tomography (CT) models of the true biomedical device in situ, such as dental implants in bone . To numerically simulate the effect of surface roughness in a FE model, Grant et al. in 2007 assumed friction coefficients to reticulate the implant microstructure to the FEA by giving a specific friction coefficient to different roughening techniques . Thus, the coefficient of friction ( μ ) for plasma-sprayed and porous-beaded implants was reported with a mean value of μ = 1.0; for sand-blasted surfaces, μ = 0.68; and for polished samples, μ = 0.4 . It is of interest to note that an increase in the coefficient of friction is believed to improve the osseo-integration and secondary stability of the implant . Equally it should be noted that an increase in the coefficient of friction reveals specific behavior to the mechanical loading . Huang et al. found that the stress state is dependent upon the surface treatment of the implant and that maximum stresses routinely occur in the cortical bone layer in close to the neck of the implant . They reported that increasing the coefficient of friction of a dental implant from 0.4 to 1 results in increased stress in the surrounding cortical bone of implant, which might increase peri-implant bone loss .
Thus a dilemma arises between the need to adopt a rough implant surface (higher coefficient of friction) that may promote osseo-integration and secondary stability, but that at the same time may also create an increased stress state at the cortical bone interface, causing loss of this bone around the implant. This controversy is further complicated by evidence suggesting that a small increase in stress in the trabecular bone of the mandible can stimulate bone adaptation and improved osseo-integration . Thus, a two-part surface treatment technique, as proposed in this investigation, may overcome the inherent limitations of a single roughness surface and provide greater benefits by simultaneously encouraging osseo-integration and primary stability whilst reducing the stress state at the cortical bone interface.
Finite Element numerical modeling is an effective tool for the analysis of the magnitude and distribution of stress fields, but the interpretation and quality of the data is strictly dependent on the accurate reproduction of all the morphological features of the real implant. Thus, the location and magnitude of the maximum stress at the bone–implant interface (BII) depends on the micro-profile and also on the accurate modeling of the implant threads. The numerical study carried out by Huang et al. could be challenged by not modeling the threads in a manner analogous to a true manufacturing process (subtractive cutting), but by the addition of fine threads onto the implant surface of the numerical model . This, previously underestimated area in implant thread design in FE computational models, may lead to a misinterpretation of the assessment of the stresses on the bone around the implant fixture. Accurate reproduction of the true manufacturing process, including the modeling of the geometry of the threads, may alter the location of maximum stress. This may be of special significance with the first thread that engages the cortical bone, which is an area of high stress location. A further hypothesis is that the use of a hybrid surface configuration on the fixture with different coefficients of friction; consisting of a coronal (cortical bone interface) polished surface and a body (trabecular bone interface) plasma treated surface will generate optimum stress distribution patterns.
The aims of this investigation are, to assess the distribution of stresses at the bone–implant interface of mandibular implants under immediate loading and the effects of surface roughness on the adjacent bone tissues. In order to evaluate the stress distribution through FEA, a sophisticated three-dimensional model for the implant–mandible bone complex has been generated. The implant model itself is carefully generated using a subtractive thread cutting process in 3D CAD modeling software. Three objectives are pursued in this study to assess the distribution of stress levels: (a) Determine the high risk regions at the bone–implant interface; (b) assess the effect of the surface roughness on the stress values; and (c) assess the effect of a multi-roughness surface technique.
2
Materials and methods
An accurate and high-resolution computational model of the bone and implant complex was created to investigate the distribution of stress within it. The model was constructed with two parts, the mandible bone and the implant; each of which are created through a complex multi-stage sequence which were subsequently combined to create the implant–bone complex. In this study, the coronal part of the implant (in contact with cortical bone) was considered as polished ( μ = 0.4), whilst the more apical component (in contact with trabecular bone) was considered either plasma-sprayed or porous-beaded ( μ = 1).
The geometrical data for the mandible bone was acquired from a computational tomography (CT) scan. The scan data were subsequently imported into an image processing software program for three-dimensional (3D) design and modeling (Mimics ® , version 13.1, Materialise Co., Ltd.) to create a shell type 3D model in a standard triangle language (STL). A solid 3D model (3D volume mesh) of the mandibular bone including cortical and trabecular bone structures; was then generated from the shell-type STL model using a 3D mechanical computer aided design (CAD) program (SolidWorks, DassaultSystèmesSolidWorks Corp; 2011). The 3D model of implant complex (fixture, abutment, connecting screw and crown) was with a design precision of 0.01 mm in a 3D mechanical computer aided design (SolidWorks, DassaultSystèmesSolidWorks Corp; 2011). The fixture was designed with the length of 11.5 mm long and width of 5 mm with square-shaped thread and a pitch length of 0.6 mm. The implant helical threads were designed and modeled in accordance with manufacturing standards in a subtractive manner. A section of the body of the mandible to receive the implant was defined by separating it from the rest of the bone to establish the boundary conditions (zone of zero displacement) with three degrees of freedom in XYZ coordinates. Fig. 1 a and b demonstrates the implant’s components in an exploded view, and the detailed modeling of the thread at the most coronal aspect. Numerical analysis was undertaken using SolidWorks Simulations (Dassault Systèmes SolidWorks Corp; 2011). The incorporated network mesh in total was 422,897 elements (tetrahedral) and the total number of nodes was 835,642. Unstructured mesh size that was variable between maximum element size of 1 mm and minimum element size of 0.1 mm was applied. Fig. 1 d, illustrates the meshed model of the mandible and implant complex.

2.1
Material properties
All the material’s properties were assumed to be isotropic, homogenous and linear elastic. The implant, abutment and fixture were considered to be made of Ti–6Al–4V, and the crown was attributed with the properties of dental porcelain. The mandibular bone including cortical and trabecular; was incorporated.
The interface between layers was assumed rigid. The elastic modulus and Poisson’s ratio for each material has been selected in accordance with the existing literature ( Table 1 ).
Material | Young’s modulus (GPa) | Poisson’ ratio |
---|---|---|
Ti–6Al–4V | 110 | 0.31 |
Porcelain crown | 82.8 | 0.33 |
Cortical bone | 14.5 | 0.323 |
Trabecular bone | 1.37 | 0.3 |
2.2
Interface condition
The current study simulated a perfect bonding (rigid) condition with no slip interface conditions between the components (fixture, abutment, connecting screw and crown). For the single-surface roughness study, the interface between the bone and the implant was modeled with three different coefficients of friction that represent implant surfaces roughened by polishing ( μ = 0.4); sandblasting ( μ = 0.68) and by plasma-spraying/porous beading techniques ( μ = 1.0) . The two-part surface implant was modeled by assigning two different coefficients of friction to the fixture. The coronal part (in contact with cortical bone) with a value of 0.4 that is equivalent to the polished surface; the lower part (in contact with cortical bone) with a value of μ = 1.0, equivalent to plasma-spraying or porous-beading.
2.3
Boundary condition and load application
Following the creation of the 3D meshes, a boundary condition was applied to simulate the natural relationship of the implant complex supported by the bone structure. Thus a boundary condition (zero displacement) for the analysis purposes was defined at all nodes of the two bucco-lingual cutting surfaces of the cortical bone that were constrained in all directions ( X , Y , and Z ) ( Fig. 1 c).
To analyze the stress state, the load was applied to the occlusal surface of the crown. For this investigation, a vertical component of 200 N and a lateral component of 20 N were applied to the occlusal surfaces of the crown ( Fig. 1 c) .
2
Materials and methods
An accurate and high-resolution computational model of the bone and implant complex was created to investigate the distribution of stress within it. The model was constructed with two parts, the mandible bone and the implant; each of which are created through a complex multi-stage sequence which were subsequently combined to create the implant–bone complex. In this study, the coronal part of the implant (in contact with cortical bone) was considered as polished ( μ = 0.4), whilst the more apical component (in contact with trabecular bone) was considered either plasma-sprayed or porous-beaded ( μ = 1).
The geometrical data for the mandible bone was acquired from a computational tomography (CT) scan. The scan data were subsequently imported into an image processing software program for three-dimensional (3D) design and modeling (Mimics ® , version 13.1, Materialise Co., Ltd.) to create a shell type 3D model in a standard triangle language (STL). A solid 3D model (3D volume mesh) of the mandibular bone including cortical and trabecular bone structures; was then generated from the shell-type STL model using a 3D mechanical computer aided design (CAD) program (SolidWorks, DassaultSystèmesSolidWorks Corp; 2011). The 3D model of implant complex (fixture, abutment, connecting screw and crown) was with a design precision of 0.01 mm in a 3D mechanical computer aided design (SolidWorks, DassaultSystèmesSolidWorks Corp; 2011). The fixture was designed with the length of 11.5 mm long and width of 5 mm with square-shaped thread and a pitch length of 0.6 mm. The implant helical threads were designed and modeled in accordance with manufacturing standards in a subtractive manner. A section of the body of the mandible to receive the implant was defined by separating it from the rest of the bone to establish the boundary conditions (zone of zero displacement) with three degrees of freedom in XYZ coordinates. Fig. 1 a and b demonstrates the implant’s components in an exploded view, and the detailed modeling of the thread at the most coronal aspect. Numerical analysis was undertaken using SolidWorks Simulations (Dassault Systèmes SolidWorks Corp; 2011). The incorporated network mesh in total was 422,897 elements (tetrahedral) and the total number of nodes was 835,642. Unstructured mesh size that was variable between maximum element size of 1 mm and minimum element size of 0.1 mm was applied. Fig. 1 d, illustrates the meshed model of the mandible and implant complex.
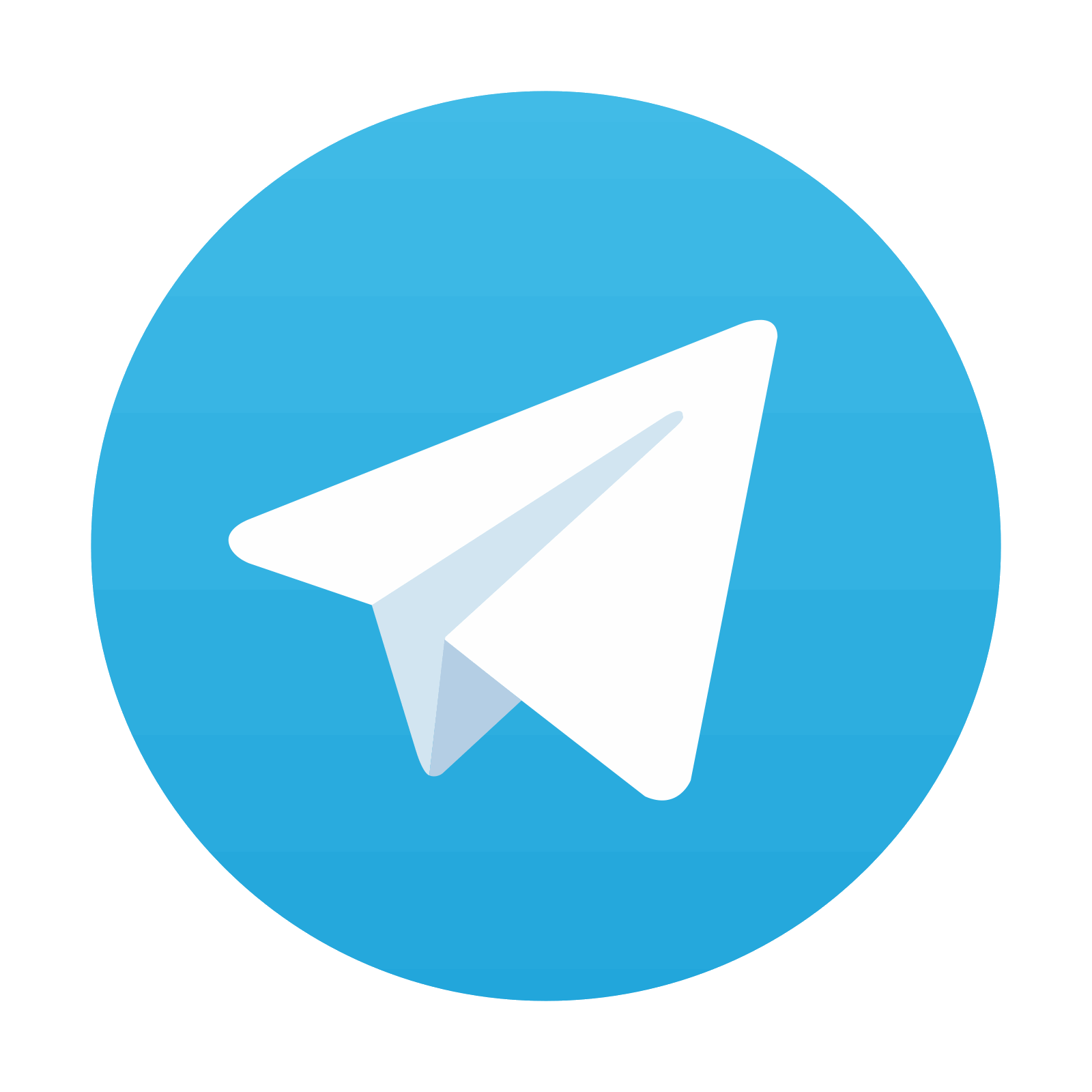
Stay updated, free dental videos. Join our Telegram channel

VIDEdental - Online dental courses
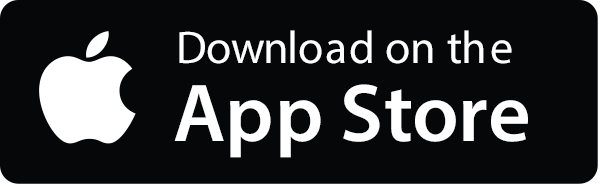
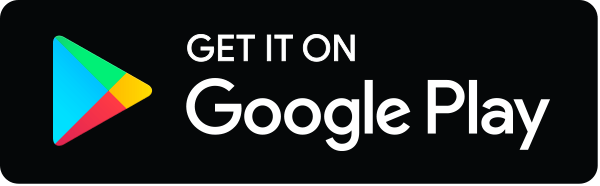
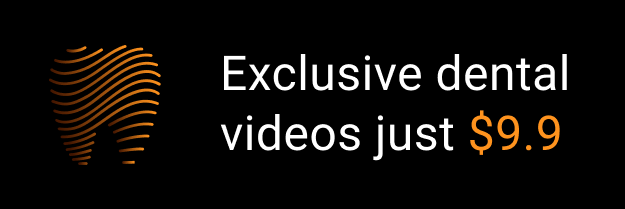