Abstract
Objectives
For posterior partial restorations an overlap of indication exists where either ceramic or resin-based composite materials can be successfully applied. The aim of this study was to compare the fatigue resistance of modern dental ceramic materials versus dental resin composites in order to address such conflicts.
Methods
Bar specimens of five ceramic materials and resin composites were produced according to ISO 4049 and stored for 14 days in distilled water at 37 °C. The following ceramic materials were selected for testing: a high-strength zirconium dioxide (e.max ZirCAD, Ivoclar), a machinable lithium disilicate (e.max CAD, Ivoclar), a pressable lithium disilicate ceramic (e-max Press, Ivoclar), a fluorapatite-based glass-ceramic (e.max Ceram, Ivoclar), and a machinable color-graded feldspathic porcelain (Trilux Forte, Vita). The composite materials selected were: an indirect machinable composite (Lava Ultimate, 3M ESPE) and four direct composites with varying filler nature (Clearfil Majesty Posterior, Kuraray; GrandioSO, Voco; Tetric EvoCeram, Ivoclar-Vivadent; and CeramX Duo, Dentsply). Fifteen specimens were tested in water for initial strength ( σ in ) in 4-point bending. Using the same test set-up, the residual flexural fatigue strength ( σ ff ) was determined using the staircase approach after 10 4 cycles at 0.5 Hz ( n = 25). Weibull parameters σ 0 and m were calculated for the σ in specimens, whereas the σ ff and strength loss in percentage were obtained from the fatigue experiment.
Results
The zirconium oxide ceramic showed the highest σ in and σ ff (768 and 440 MPa, respectively). Although both lithium disilicate ceramics were similar in the static test, the pressable version showed a significantly higher fatigue resistance after cyclic loading. Both the fluorapatite-based and the feldspathic porcelain showed equivalent initial and cyclic fatigue properties. From the composites, the highest filled direct material Clearfil Majesty Posterior showed superior fatigue performance. From all materials, e.max Press and Clearfil Majesty Posterior showed the lowest strength loss (29.6% and 32%, respectively), whereas the other materials lost between 41% and 62% of their flexural strength after cyclic loading.
Conclusions
Dental ceramics and resin composite materials show equivalent fatigue strength degradation at loads around 0.5 σ in values. Apart from the zirconium oxide and the lithium disilicate ceramics, resin composites generally showed better σ ff after 10,000 cycles than the fluorapatite glass-ceramic and the feldspathic porcelain. Resin composite restorations may be used as an equivalent alternative to glass-rich-ceramic inlays regarding mechanical performance.
1
Introduction
The guidelines for the restoration of small to medium-size defects in the posterior region of teeth present an overlap of indications, where both direct or indirect techniques are applicable. Apart from empirical criteria, little relevant evidence is available to the dentist, which could be used as a solid principle for the choice of one treatment option over the other.
The terms “direct” and “indirect” restorations relate mostly to the in situ placement of resin composites and to the machined- or laboratory-made ceramics, respectively. Both alternatives show technical advantages and disadvantages. Tissue removal during preparation for direct resin composite follows minimally invasive conducts, pleasing patients and dentists. Resin composites however contract, and in situ polymerization may inflict temporary flexural stresses on damaged cavity walls and prompt premature cracking of thin enamel borders. Resin composite restorations are also more technique sensitive, color unstable and less wear resistant than their ceramic restoration counterparts. In turn, economical aspects are sure to make direct restorations with resin composites an attractive option to costly indirect processes and materials.
This balance of pros and cons contain most of the factors that are usually taken into account by dentists to deal with this conflict. However, these are hardly the factors that have weight in determining the success rate of a type of restoration. Instead, clinical studies show that failure of small to medium-size posterior restorations mostly happens in one of two ways . The first one is biological and is characterized by the infiltration of bacteria through the restorations margins causing tissue degradation (secondary caries). The other is mechanical and results in partial or complete fracture of the restoration. These two factors are not related to the technique itself, but rather to the materials in use. Secondary caries, for example, may depend on the caries risk factor of the patient , but it is ultimately determined by the bonding effectiveness of the bonding/luting agent. Likewise, the fracture process depends on the subjected load, but it is primarily governed by the mechanical properties of the material.
That supports the study of the mechanical performance of resin composites and dental ceramics for creating criteria that are more clinically relevant for determining treatment indications. Mostly, mechanical properties such as elastic modulus, flexural strength and fracture toughness are assessed under static loading conditions for the initial characterization of materials. In this regard, substantial differences are observed between resin composites and dental ceramics. The latter are stiffer, showing elastic moduli between 65 GPa (feldspathic and glass-ceramics) and 250 GPa (alumina- and zirconia-based ceramics) , whereas the interval 8–15 GPa is common for most resin composites . Ceramics may be as strong as 900 MPa in bending for pure crystalline materials , whereas glass-rich ceramics, such as feldspathic- and leucite-based, show flexural strengths similar to resin composites (70–130 MPa) . The values of fracture toughness in resin composites (1–2 MPa√m) , however, surpass those of glass-rich ceramics (0.7–1.5 MPa√m) used for inlays, onlays and veneering of infrastructures. For lithium disilicate-based glass-ceramics an increase in fracture toughness (∼3 MPa√m) is observed despite the relatively high amount of glass (30%) due to toughening mechanisms induced by the microstructure . A complete elimination of the glass phase leads to high values of toughness in zirconia and alumina ceramics (5–7 MPa√m) .
The above-mentioned parameters are particularly relevant during overloading events that lead to fast fracture. This scenario is however not that representative of clinical failures . For applications where the loading conditions are rather repetitive and subcritical, such as those experienced in the mouth, studies that characterize the fatigue behavior and strength degradation of restorative materials might offer a higher predictive potential . In resin composites, for instance, properties derived from fast fracture conditions have shown little association to their fatigue resistance under cyclic loading in vitro . For ceramic restorations, in vitro fatigue experiments have shown to be good predictors of their in vivo survivability .
Under cyclic loading, the degradation of strength can be directly assessed by measuring the crack advance in relation to the range of stress intensity factor ( K ) applied. Using the Paris law relationship during Regimen II, subcritical crack growth susceptibility parameters ( n ) can be derived. The tracking of crack growth in materials presenting low fracture toughness and low R-curve behavior can be problematic, with obtained n values showing a high variation among studies. For glass-rich ceramics the literature reports values between 3.7 and 54.6 , and between 5.2 and 70.5 for resin composites. A more practical method for ceramic materials derives slow crack growth susceptibility parameters ( n dyn , for dynamic loading) indirectly using a range of stressing rates. Unfortunately, due to the substantial viscoelastic response of resin composites at low strain rates, the latter method is suboptimal for comparing the fatigue resistance of resin-based materials against highly brittle dental ceramics.
Aiming a practical comparison of the fatigue resistance of resin composite and dental ceramic materials, the present study used a phenomenological approach that calculates the residual flexural fatigue strength for a defined loading challenge. By selecting different compositions among currently marketed resin composites and dental ceramics, discussions on the clinical applications of these materials were conducted based on their mechanical performance.
2
Materials and methods
2.1
Materials
Five currently commercially available resin composite materials for direct (four materials) and indirect (one material) restorations were selected for testing. In Table 1 details about their filler configuration and matrix composition can be found. This selection of composites included mainly Bis-GMA-based composites with a filler content between 55 and 82 vol%, one of them containing prepolymerized fillers (Tetric EvoCeram, Ivoclar-Vivadent, Liechtenstein) and one machinable indirect composite (Lava Ultimate, 3M ESPE, USA).
Composite | Manufacturer | Filler content (vol%) | Filler configuration | Matrix composition | Lot |
---|---|---|---|---|---|
Lava Ultimate | 3M ESPE, USA | 80 | 20 nm silica particles; 4–11 nm zirconia particles; 0.6–10 μm nanoparticle clusters | Bis-GMA, Bis-EMA, UDMA, TEGDMA | 602 |
Clearfil Majesty Posterior | Kuraray, Japan | 82 | 0.5–10 μm particles; 20 nm particles | Bis-GMA, TEGDMA, hydrophobic aromatic dimethacrylates | 00111B |
Grandio SO | Voco, Germany | 73 | 0.5–3 μm particles; 20–40 nm nanoparticles | Bis-GMA, Bis-EMA, TEGDMA | 1207326 |
Tetric EvoCeram | Ivoclar-Vivadent, Liechtenstein | 55 | 0.5 μm mean particle size; 5–50 μm prepolymerized fillers containing 0.4–0.7 μm fillers | Dimethacrylates | P67534 |
CeramX Duo | Dentsply, Germany | 57 | 1.1–1.5 μm glass particles; 10 nm particles | Bis-GMA, UDMA, TEGDMA | 1211000259 |
Five ceramic systems were selected to encompass different compositions (crystalline structure and glass content) and clinical indications for inlays or onlays ( Table 2 ). Reinforced systems that can be used as infrastructures or monolithically included a zirconium dioxide ceramic (IPS e.max ZirCAD, Ivoclar-Vivadent) and a lithium disilicate glass-ceramic in two forms: a machinable block (IPS e.max CAD, Ivoclar-Vivadent) or a pressable variant (IPS e.max Press, Ivoclar-Vivadent). Glass-rich ceramic systems with restricted indications for use in the monolithic form or as veneers included a fluorapatite glass-ceramic powder for use with the layering technique (IPS e.max Ceram, Ivoclar-Vivadent) and a machinable color-graded feldspathic porcelain block (Vitablocs Trilux Forte, Vita, Germany).
Ceramic | Manufacturer | Crystal structure | Glass phase (wt%) | Range of indications | Lot |
---|---|---|---|---|---|
IPS e.max ZirCAD | Ivoclar-Vivadent, Liechtenstein | Yittrium-stabilized zirconium dioxide crystals | 0 | Single-unit to long-span infrastructures or monolithic structures | L47695 |
IPS e.max CAD | Ivoclar-Vivadent, Liechtenstein | 0.2–2 μm lithium disilicate crystals | 60 | Inlays, onlays, veneers, anterior and premolar crowns, veneering of infrastructures | P19972 |
IPS e.max Press | Ivoclar-Vivadent, Liechtenstein | 3–6 μm lithium disilicate crystals | 30 | Inlays, onlays, anterior and posterior crowns, 3-element bridges in the anterior and pre-molar regions, veneering of infrastructures | J18904 |
IPS e.max Ceram | Ivoclar-Vivadent, Liechtenstein | 0.1–5 μm fluorapatite crystals | up to 90 | Inlays, laminate veneers, veneering of infrastructures | M04720 |
Vitablocs Trilux Forte | Vita Zahnfabrik, Germany | 4 μm feldspat crystals | 80 | Inlays, laminate veneers, anterior crowns and veneering of infrastructures | 12500 |
2.2
Specimen preparation
2.2.1
Resin composite specimens
Forty bending bars (2 mm × 2 mm × 25 mm) were produced for each material using a tungsten carbide/glass mold under calibrated conditions of 23 °C and 50% humidity. The specimen fabrication followed the manufacturers’ recommendations and ISO 4049 standard. The materials were inserted in the mold in one previously weighted increment, and light-cured with five overlapping spots of ∅ = 8 mm on both top and bottom with a commercial light-curing unit (Elipar ® Trilight, 3M ESPE, Germany) with an output intensity of 800 mW/cm 2 for 20 s at each spot. After removal from the mold, the bottom of the specimens was polished with silicon carbide paper down to 4000 grit to remove surface flaws that could influence strength values . For the indirect material Lava Ultimate, bending bars of same dimensions were supplied by the manufacturer and further polished following the procedures used for the other tested materials. The specimens were stored in distilled water at 37 °C for 14 days before mechanical testing.
2.2.2
Ceramic specimens
From the materials that were supplied in machinable blocks (IPS e.max ZirCAD, IPS e.max CAD and Vitablocs Trilux Forte), bar specimens were cut using a low-speed saw and a diamond-coated copper disk (Buheler, USA) under water lubrication. Bars of IPS e.max CAD were further crystallized over a crystallization tray (Ivoclar-Vivadent) in a furnace (Vita Vacumat 4000, Vita) at a maximum temperature of 850 °C according to the program provided by the manufacturer. Zirconium dioxide bars of IPS e.max ZirCAD were sintered in a high-temperature furnace (Linn EVA-1700, Germany; 200 °C/h heating rate) for 2 h at the maximum temperature of 1530 °C and cooled overnight. The fabrication of IPS e.max Press bar specimens followed the “lost-wax” technique, where PMMA bars were invested (PressVest, Ivoclar-Vivadent) and molten to create a space where the pressable material was subsequently injected (EP 500 furnace, Ivoclar-Vivadent). The bars were divested and sandblasted with 50 μm glass particles at 2 bar pressure ( Fig. 2 a).
After bar fabrication steps, specimens from all the ceramic materials were polished in water with SiC paper 1200 grit on all sides and further down to 4000 grits at the side subjected to tension. Final dimensions of ceramic specimens were the same as for the resin composite specimens.
The specimens from IPS e.max Ceram were produced using the layering technique, in which a powder-distilled slurry was poured into a detachable custom-made Plexiglax mold, pressed and dried with absorbent paper. The ceramic powder plate was removed from the mold and sintered over a refractory tray in a furnace (Vita Vacumat 4000, Vita) at a maximum temperature of 750 °C following the sintering program provided by the manufacturer. The resulting sintered ceramic plate presented final dimensions of 27 mm × 21 mm × 3 mm. The plates were subsequently ground with a diamond wheel in a grinding machine (MPS 2 120, G&N, Germany) to obtain 2.1 ± 0.1 mm-thick plates with perfect parallel sides. Further polishing steps were undertaken with SiC papers down to 1200 on all sides and up to 4000 grits on the side subjected to tension. The polished plates were then sectioned in multiple 2 mm-thick bars using a Micro Water-Jet cutting machine (Womajet F3, Daetwyler, Switzerland) operating at 6000 bar pressure and 1000 m/s water jet speed ( Fig. 2 b ). The use of a high-pressure abrasive–water mixture cutting procedure allowed for the structural preservation of the material without further alterations due to heating.

The specimens from all ceramic materials were stored in distilled water for 14 days at 37 °C before mechanical testing.
2.3
Initial flexural strength ( σ in ) and flexural fatigue strength ( σ ff ) testing
For the initial flexural strength ( σ in ) measurements, 15 specimens per material were loaded in 4-point bending ( Fig. 1 a) at 0.75 mm/min in a universal testing machine (Z2.5, Zwick, Germany) using the relation:
σ in = 3 P d w b 2
where P is the maximum load at failure, d is the distance between the midpoint of the lower supports and the upper supports (5 mm), w the width and b the height of the specimen. The initial strength data was further analyzed according to a two-parameter Weibull distribution in terms of shape ( m ) and scale parameters σ 0 and σ −2.97 . The m modulus describes the scatter in stress to failure, and parameters σ 0 and σ −2.97 indicate their magnitude and are given for a failure probability of 63.2% and 5%, respectively. The Weibull modulus m , the characteristic strength σ 0 and their respective 95% confidence intervals were corrected by a factor corresponding to the number of specimens ( n = 15), according to the European standard EN 843-5 .

The flexural fatigue strength ( σ ff ) of the composite and ceramic materials was determined for 10 4 cycles under equivalent test conditions at a frequency of 0.5 Hz ( n = 25). The “staircase” approach method was used for the fatigue resistance evaluation. For each cycle, the stress alternated between 1 MPa and maximum applied stress ( σ app ). Tests were conduced sequentially, with σ app in each subsequent specimen being increased or decreased by a fixed increment of stress, according to whether the previous 10 4 cycle runs resulted in failure or survival. The first specimen was tested at σ app = 0.5 σ in . All tests were carried out in distilled water at 37 °C. The σ ff and standard deviation (SD) were determined using Eqs. (2) and (3) , respectively;
σ ff = X 0 + d ∑ i n i ∑ n i ± 0.5
SD = 1.62 d ∑ n i ∑ i 2 n i − ( ∑ i n i ) 2 ( ∑ n i ) 2 + 0.029
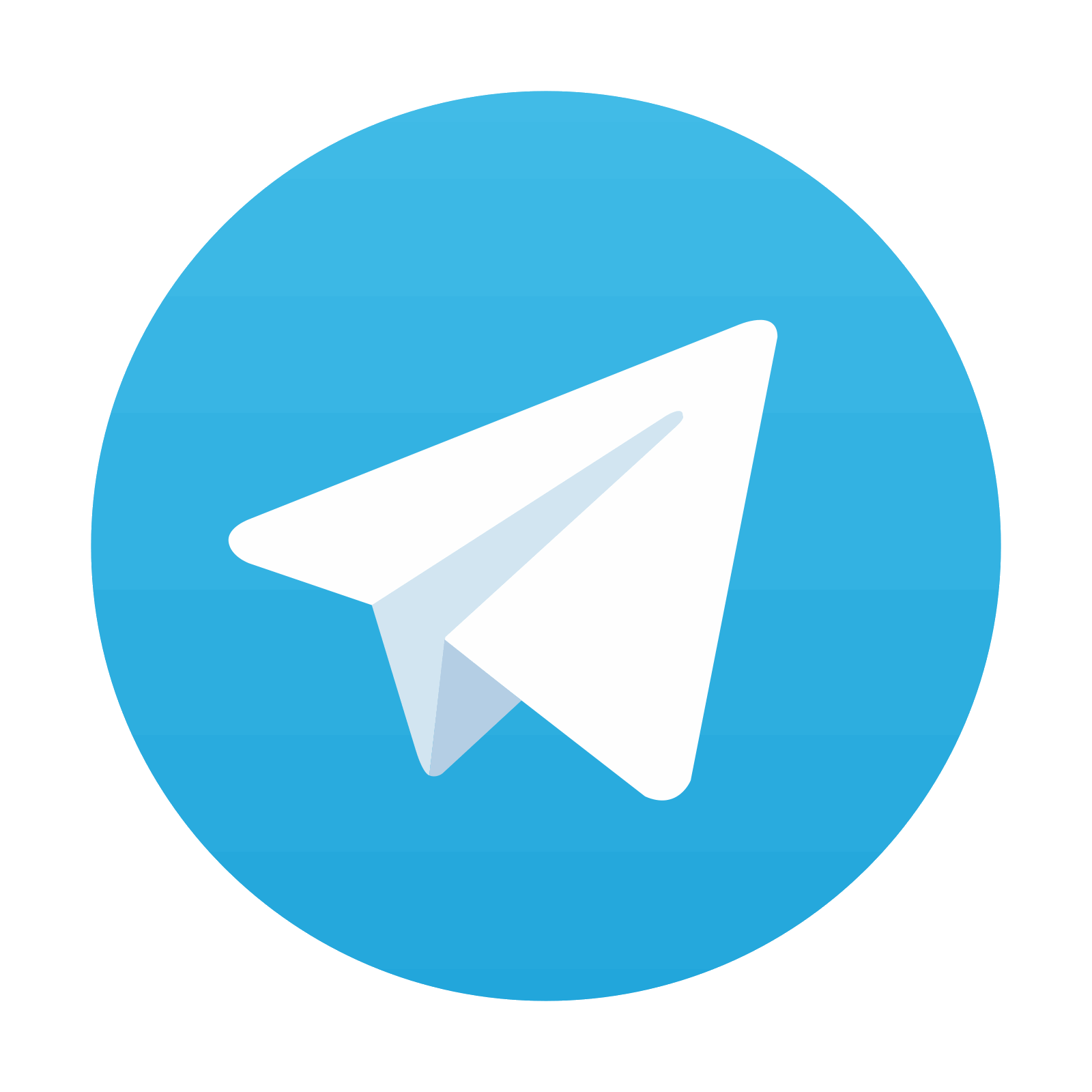
Stay updated, free dental videos. Join our Telegram channel

VIDEdental - Online dental courses
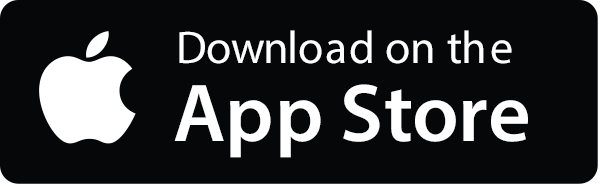
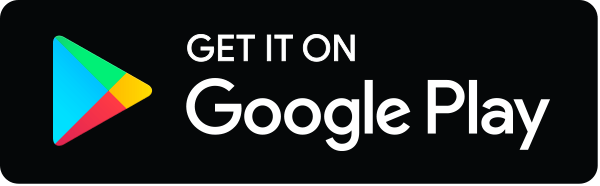