1
Introduction to Oral and Craniofacial Tissue Engineering
Introduction
Oral and craniofacial tissues are important in several physiological functions such as mastication, speech, facial aesthetics, and the most important in the quality of health in life. In this fundamental role, teeth are essential to these functions relying on its unique combination of hard tissues—including enamel, dentin, root cementum and alveolar bone and soft tissues—including periodontal ligament, gingiva and dental pulp (Orsini et al. 2018a, b). The development of all organs which form from the ectodermal and endodermal sheets lining the embryo is regulated by the communication between the epithelium and underlying mesenchyme. Teeth are unique, greatly specialized organs, in which studies of classic tissue recombination in the developmental biology field established that the tooth shape, dental cells and tissues, takes place through a strict series of well-defined regulated stages that involve this kind of communication (Luukko and Kettunen 2016). This process in tooth development is characterized by complex reciprocal interactions between the epithelial and mesenchymal tissue, which occur in a stepwise process classically described, from early to late as Lamina, Bud, Cap and Bell stages (early and late stage), in which each phase is discernible by specific histomorphological and cellular features (Yildirim et al. 2011; Mitsiadis and Harada 2015; Thesleff 2014). In recent years, with a better understanding of molecular biology and cell-tissue signaling, the process of tooth development could be broadly described as a sequential differentiation process mediated by the conserved signaling pathways, such as FGF, BMP, Hedgehog, EDA, and Wnt, where the basal cells of the dental lamina (dental epithelial tissue) undergo proliferation and form a horseshoe-shaped band that invaginates into the underlying mesenchymal tissue (this process is called epithelium invagination). The mesenchymal tissue, derived from neural crest cells, proliferated and differentiated to ameloblasts for depositing the enamel tissue, meanwhile mesenchymal tissue responds to dental epithelial signaling beginning with the process of differentiation into cementoblasts, periodontal ligament, odontoblasts and other dental pulp cells (including neurons, endothelial cells and fibroblasts) (Caton and Tucker 2009; Balic 2018; Xiao and Nasu 2014). Thus, tooth-specific tissues originating from the two principal sources gives the complete anatomy of soft and hard tissues in teeth, including dentin, dental pulp, alveolar bone and periodontal ligament.
In dentistry, it is well known that in humans these soft and hard tissues could be lost due to damage of tissue by trauma, dental caries, periodontal disease or a variety of genetic disorders that combined with age could lead to suffering a physical and mental dramatic event that compromises an individual’s self-esteem and quality of life (Batista et al. 2014; Kassebaum et al. 2014). It is, therefore, necessary to develop innovative approaches for the repair/regeneration of damaged or missing alveolar bone and dental tissues (Caton et al. 2011). Recently, with the recognition of the molecular and cell biology based on the development of dental tissues structure, more and more efforts have been focused on applying the knowledge gained to design therapies to promote the dental tissues regeneration because modern dentistry is not limited to maintenance of dentition but has many subspecialties encompassing diagnosis and treatment of conditions affecting the oral and maxillofacial structures. Thus, regeneration of lost dental tissue by the rehabilitation of patients has been the ultimate dream of every clinician and dental healthcare researcher. Due to the unique, diverse role of the teeth and associated structures, there has been a sustained effort to replace the missing dentition over many centuries. This effort could be seen by several examples of proposed approaches to engineer biological dental tissues including tissue engineering scaffolds; cell-tissue recombination, gene-manipulated cell for improve tooth regeneration, dental mesenchymal stem cells co-cultures, 3D tissues culture strategies and 3D scaffold design by polymer printing, bioprinting and CAD-CAM technology (Moioli et al. 2007; Jahangirian et al. 2018; Saratti et al. 2019; Werz et al. 2018).
This chapter describes the strategies for the regeneration of oral and craniofacial tissues. First, to address the principles of the specific area related to dental tissue engineering, second to describe the components of the extracellular matrix and what strategies are used to try to mimic the natural extracellular matrix of tissue structures by using of scaffolds and, finally, what are those strategies that are being used to try to understand how to achieve the regeneration of the oral and craniofacial complex.
Dental Tissue Engineering
Oral and craniofacial tissues have a limited ability to correctly auto regenerate when the original tissue integrity has been severely damaged. Moreover, this limitation on the structural integrity of the damaged tissue is because of several common conditions requiring craniofacial and dental treatment including caries pulp, odontogenic infections, periodontal disease, tooth impactions, tissue dysfunction and malocclusion disorders are constant challenges in the dental health area (Gurtner et al. 2008; Duailibi et al. 2008). Moreover, with the high expectation of life, and with the increasing number of cases of dental pathologies related to the traumatic, inflammatory and neoplastic origin, congenital anomalies and degenerative diseases affecting the principle of oral and craniofacial structures, a new area that is getting relevant as a strategy to address these problems and developing new biological therapies to face this wide range of dental issues is dental tissue engineering.
Dental tissue engineering is a multidisciplinary approach that tries to the restore, maintain and enhance oral tissue function along with diagnostic and clinical applications. Dental tissue engineering works to combine the basic principles of physics, chemistry, tooth development, cell biology, nanotechnology, regenerative medicine and engineering to create a logical strategy approach to generate functional oral tissues at cellular and molecular levels that closely match with the physical and mechanical properties of naturally formed oral and craniofacial tissues (Rai 2015; Bossu et al. 2014). However, dental tissue engineering could be summarized in three central components of this multidisciplinarity as specific source of living cells, because one of the biggest challenges in the repair/regeneration strategies is what kind of cells must be used? Or what kind of specific phenotype of source cells must be isolated? Considering that oral and craniofacial tissue have distinct functions and coupled with this problem, also a different extracellular matrix that could be regarded as soft or hard, implies the uses of biomaterials as an artificial structure. This artificial structure also assumes the understanding of how to develop new materials that meet the physicochemical, structural and mechanical properties to instruct cellular sources to respond to deposit a specific cellular tissue, in which the bioactive signals (peptides, growth factors or genes) finally enter that have to be integrated in the artificial structure to give biological cues and regulate cell functions (Fig. 1.1).
There is no doubt that in dental tissue engineering cells play a central role in developing innovative strategies for repair/regeneration of the oral and maxillofacial region. But today not all kind of cells can be used as a source for this purpose, only specific cells that are immature, unspecialized, that have self-replication and conserve their potency and cell plasticity to differentiate into a vast variety of cells populations including dental tissue have gained attraction and are particularly important for developing tissue engineering strategies in this area. These unspecialized cells called dental mesenchymal stem cells could be identified and isolated based on their adherence to tissue-culture-treated plastic when being cultivated in vitro, for their rapid expansion, for showing a fibroblastic morphology, and for having a positive signal and expressing CD 105, CD73 and CD90 surface markers and lacking the expression of CD45, CD34, CD 14 or CD 11b, CD79a or CD 19 and HLA-DR surface molecules. Dental mesenchymal stem cells isolated from different tissues, for example from oral mucosa, pulp, periodontal ligament and gingiva represent an excellent source for dental cell therapy and regeneration of damaged oral and maxillofacial tissues. This option as a source is because they display multipotency when specific factors are used for inducing the differentiation showing the capacity to give rise to osteo/odontogenic cells, chondrocytes, adipocytes, neuronal cells, muscle cells, cardiomyocytes, endothelial cells, hepatocyte-like cells and islet-like cells (Bartold and Gronthos 2017; Han et al. 2014; Ge et al. 2012; Egusa et al. 2012).

Figure 1.1. Schematic representation of the three principal components of dental tissue engineering involved in the challenge for designing new strategies for tissue repair/regeneration.
A detailed chapter on this topic, will focus on mesenchymal stem cells isolated from a different source of dental tissues and also address the subject of how the mesenchymal stem cells could be used as a cell-based therapy with its potential clinical applications. Moreover, these days new cell-based approaches for enhancing the cell response on regeneration strategies that deal with replacing the defective genes with their correct analogues to produce functional proteins will be addressed in the chapter focused on gene therapy that definitely becomes a potential and promising future treatment modality of a number of diseases especially affecting the oral and craniofacial tissues.
As a second point on dental tissue engineering, important elements for tissue regeneration and for the success and efficacy of the knowledge in the cell therapy not only involve genes and dental mesenchymal stem cells but also designing appropriate scaffolds that should be biocompatible, non-toxic, under biologically safe degradation and eventually resorbed by remodeling the new tissue, allowing it to acquire similar mechanical properties and withstand mechanical forces (Zafar et al. 2015).
The importance of this kind of artificial architecture or scaffolds will be addressed in more detail in various chapters of the book that try to focus on topics such as biodegradable polymers, calcium phosphates and bioactive glasses, metal organic and hybrids, composite materials and injectable scaffolds on the applications on the regeneration strategies of oral and craniofacial tissues. Moreover, an interesting point in the synthesis of biodegradable scaffolds is that surface chemistry cues presented in the form of micro- and nanoscale topographies are significant in regulating the behavior of cells because it must be instructive and this topic will be addressed in the chapter related to biomimetic approaches for the design and development of multifunctional bioresorbable layered scaffolds for dental regeneration.
Thus, the cell instructive property implies that topography of the scaffolds gives a specific signal that allows functionality to trigger the cascade of events and also the architecture of the scaffold has to mimic the scale of the extracellular matrix creating an artificial microenvironment that improve and may direct the differentiation of stem cells towards a precise fate and function leading to regeneration (Choi et al. 2018; Darnell et al. 2018; Liu et al. 2018). Thus, nanosurface scaffolds such as nanoparticles, nanoceramic, nanofibers and nanocomposites have gained increasing interest in dental tissue engineering. This larger interest is because topography including pore size and porosity also have a direct influence on control stem cell behavior as cell attachment, cell morphology, cell proliferation, cell differentiation that allow to guide tissue repair/regeneration (Hettinger et al. 2009; Mansouri and SamiraBagheri 2016; Skoog et al. 2018).
The mechanism by which the topography exerts its effect on stem cell response is by altering focal adhesion assembly and cytoskeletal stress, resulting in adaptive gene- and protein-level changes (Kolind et al. 2014). Thus, the surface topography features of sub-micrometers to 10 µm in size act on the actin cytoskeleton, whereas features of ten to hundreds of nanometers act on integrin receptors (Miyoshi and Adachi 2014). However, the cell-surface interaction regarding the link between topographical cues and changes at proteomic, genomic and epigenomic levels are not completely understood.
Furthermore, topography of the scaffold could also be relevant for hydrophilicity and for specific protein adsorption, as shown by the selective take up of proteins relevant for cell attachment, such as fibronectin and vitronectin, on fibrous meshes with nanoscale fiber diameters (Bai et al. 2018).
Indeed, since most types of mesenchymal stem cells are dependent on anchoring and could die if there is not a favorable cell adhesion by the surface instruction of the scaffold; the search for functionalization of the scaffold for enhancing a favorable cell—material interaction are paramount for inducing a specific signaling of cellular respond at the cell—biomaterial interface. This could be achieved by integrating bioactive signals as cell adhesion peptides or growth factors or by specific adsorption of endogenous extracellular matrix proteins, as functionalization of the surface scaffold by the different methods to synthesize the scaffolds as solvent evaporation, salt-leaching, molecular self-assembly, gas foaming, phase separation, emulsification/lyophilization, 3D rapid prototyping and electrospinning, for help to regulate cell function.
Finally, the third point in dental tissue engineering, signaling biomolecules as growth factors are the choice for functionalization because these proteins activate the cellular communications network and influence functions, such as cell adhesion, cell proliferation, matrix deposition and differentiation of tissues. Also, growth factors have been shown to play a key role on the induction of fracture and wound healing, formation and repair of all craniofacial tissues and there are at least six growth factors that could be involved in oral and craniofacial tissue engineering that have been used in vitro and in vivo as Platelet-Derived Growth Factor (PDGF), basic Fibroblast Growth Factor (bFGF), Insulin-like Growth Factor (IGF), Transforming Growth Factor beta (TGFb), Vascular Endothelial Growth Factor (VEGF), and Bone Morphogenetic Proteins (BMPs) (Mandla et al. 2018). Moreover, structural fibrous protein of the extracellular matrix provides adhesive ligands such as collagen, elastin, keratin, laminins, fibronectin and vitronectin that direct cell function and could allow a more precise regulation of cell function and tissue formation (Cruz-Maya et al. 2018).
Extracellular Matrix Mimicry Dental Tissue Engineering Scaffolds
The loss of tissues due to trauma, disease or congenital anomalies is a health care issue. Therefore, the development of materials that could be implanted or incorporated into tissues to regain function is of utmost importance. The global market for biomaterials was valued at US $ 66.2 billion in 2015 and is likely to expand to 14% by 2020 (Nagrath et al. 2018). A large part of this market is centralized on mineralized tissues such as orthopedic and craniofacial/dental implants. In dentistry, tooth loss is not seen as an aesthetic or psychological problem; this is definitively a problem with serious physiological consequences. Tooth loss should be considered as an imbalance in the masticatory system. Edentulism can lead directly to impairment, functional limitation, gum damage and alveolar bone resorption (Polzer et al. 2010). For many years, autologous implants were considered the gold standard However, this requires a more significant discomfort for the patient. Here we try to describe the recent advances in dental tissue engineering which will likely produce alternatives to autogenous bone grafts that may well exceed existing clinical outcomes and replace traditional indications for its use (Misch 2010).
The alternative to the gold standard toward the fundamental understanding of structure-function relationships in normal and pathological oral and craniofacial tissues could be the development of biological substitutes to restore, maintain or improve tissue function (O’Brien 2011). This biological substitute named biomaterial scaffold could be defined as either naturally occurring materials in living tissues or materials designed, fabricated, tested, applied and synthetic to replace, and repair oral and craniofacial tissues, which can perform, enhance or replace a specific biological function (Stupp and Braun 1997; Ratner et al. 2013). Moreover, these biomaterial scaffolds should be able to interact with cells to perform their function with an appropriate host response in a specific clinical application (Williams 2014).
In dental tissue engineering, the biomaterial scaffold basically acts as a template for cell growth and recolonization of injured tissue areas as a conductor tissue growth Scaffolds not only provide a mechanical support, but they also may present biochemical signals like growth factors to encourage cell attachment, migration onto or within the scaffold, cell proliferation, cell differentiation and modulate cell behavior (Hutmacher 2001). Some scaffolds are frequently seeded with stem cells to accelerate cell recruitment and homing (Hussey et al. 2018). Scaffolds are made from several materials like polymers, metals, ceramics or composites. Therefore, before making the selection of the material for the scaffold design, it is necessary to understand the mechanical properties—structure relationships of the tissue to replace. The scaffolds are designed to replace a wide variety of soft tissues such as skin, tendons, blood vessels, among others, and they are generally composed of synthetic or natural polymers. Replacement of mineralized tissues such as craniofacial tissues, bone and tooth are usually designed scaffolds built up from metallic, ceramics, polymers and composites materials and must be designed to be permanently implanted. Nevertheless, long-term complications such as stress shielding, wear debris, loosening and mechanical or chemical breakdown of the material itself, encouraged scientists to develop new materials. Scaffolds, in addition, must provide high porosity, high surface area, structural strength, three-dimensional micro- and nano-structure, and biodegradability if needed (Vats et al. 2003). However, an important key to design a cellular scaffold and it should be pointed out as essential for the biological response is if the scaffold could replicate or mimic the extracellular matrix properties. To understand the challenge of replicating the extracellular matrix, of oral and craniofacial tissues we will briefly explain the extracellular matrix functions and mention their most representative members.
Extracellular Matrix
The extracellular matrix is a non-cellular structure present in all vertebrate tissues. The origin of multicellularity in metazoans is intimately related to the development of the extracellular matrix. The evolutionary transition from unicellular to multicellular organisms was a fundamental change in the history of living beings on Earth. At the beginning of cellular cooperation, the cells were able to work in a cohesive way to perform more complex and sophisticated tasks; this was due to the emergence of the proteins secreted by the cells and their particular structural arrangement (Czaker 2000).
Extracellular matrix dictates several cellular functions as migration, differentiation, proliferation, morphogenesis, growth, survival and maintains tissue homeostasis (Frantz et al. 2010; Bonnans et al. 2014). Extracellular matrix maintains a highly organized three-dimensional heterogenous fibrillar network structure with tissue-specific composition and topology, that provides an indispensable physical scaffolding for the cellular constituents and is under constant and highly coordinated remodeling throughout the entire lifetime, in accordance with physiological or pathological conditions (Theocharis et al. 2016).
The extracellular matrix is composed of water and macromolecules like fibrous proteins and proteoglycans (Frantz et al. 2010). The most abundant proteins in the extracellular matrix are collagens, elastins, fibronectin, tenascin, laminin and fibrillins. Besides, extracellular matrix contains significant contents of polysaccharides as glycosaminoglycan (GAG) chains covalently attached to a specific protein core (PG-proteoglycans), except for the hyaluronic acid (Rozario and DeSimone 2010). The GAG chains are generally long linear and negatively charged with disaccharide repeats. PG make up a hydrogel due to their extended conformations and remarkably hydrophilic character. This cross-linked biopolymer gel is needed to hold up high compressive forces (Kwansa et al. 2014). The PG more abundant in the extracellular matrix are perlecan, syndecan, decorin, aggrecan, glypican and lumican. The three principal families are Small Leucine-Rich Proteoglycans (SLRPs), modular proteoglycans and cell-surface proteoglycans (Schaefer and Schaefer 2010; Kresse and Schönherr 2001).
Collagens are the most abundant family of proteins in the animal kingdom with at least 45 different collagen genes that code for collagen polypeptides, and account for up to 30% of total protein mass present in the interstitial of the extracellular matrix (Rozario and DeSimone 2010). Moreover, collagen fibrillar and non-fibrillar proteins that can be assembled into supramolecular structures, to give organization and rigidity to the extracellular matrix. The mechanical properties of the extracellular matrix can be determined by the organization, distribution and density of the collagen fibers in the tissues (Birk and Brückner 2011). Mutations in the genes of collagen can be fatal in embryonic development, even embryos lacking collagen I that reach late stages of development suffer from abrupt rupture of the aorta due to lack of tissue compliance and it is striking that one collagen type can predominate overwhelmingly in tissues that are extremely diverse (Löhler et al. 1984).
All collagen members share common features. The collagen proteins have at least one triple alpha helix collagenous domain (COL), which is a rod-like structure to provide stiffness and the content or number of repetitions of this COL domain depends on the specific type of the collagen. The alpha helix chains are supercoiled to form a triple helix. The COL domain frequently has a triplet sequence Gly-X-Y, where Gly corresponds to the glycine residues and generally by steric constraints occupies the central positions in the triple helix. Meanwhile, X and Y can be any amino acid but frequently are found in these positions to proline and hydroxyproline, respectively. Hydroxyproline residues are essential to triple helix stability (Birk and Brückner 2011).
In the extracellular matrix, there are mainly two types of elastic fibers, chemically and morphologically different: elastins and microfibrils. Elastin fibers comprise approximately 90% of all of them. Elastin fibers provide the properties of resilience and elasticity to the tissues preventing deformation when they are under repeated stretching (Mithieux and Weiss 2005). However, the elastin fibers stretch is limited due to its close structural association with collagen fibers. Poles apart to collagen family that is encoded by several genes, elastin is encoded by a single gene in mammals and secreted to the extracellular medium as a monomer with a molecular weight of 60–70 kDa, called tropoelastin (Frantz et al. 2010). In the extracellular space, the secreted tropoelastin molecules are processed to elastin fibers; this process is catalyzed by a member of the lysine oxidase family, > 80% of the lysine residues form covalent cross-links between and within the elastin molecules. The arrangement and size of elastin fibers vary according to the tissue, but elastin fibers are associated with different proteins such as fibulins and fibrillins, which are essential to preserving the integrity of elastin fibers (Muiznieks and Keeley 2013).
Another essential fibrous protein in the matrix for playing a crucial role in the organization of the interstitial of the extracellular matrix and for interactions with cells is fibronectin. Fibronectin is directly related to central cellular events related to the matrix, such as cell adhesion, migration, growth and differentiation. Fibronectin is a protein made up of a dimer that is held together by a pair of disulfide linkages in the carboxyl terminus, and each monomer has a molecular weight around of ~250 kDa. Similar to elastin, fibronectin is a protein encoded by a single gene. However, fibronectin may occur in different versions due to alternative splicing, generating up to 20 known isoforms. Fibronectin can be stretched several times over its resting length by a neighboring cellular traction force. Owing to the tensile strength over fibronectin, it undergoes to conformational changes to expose integrin-binding sites recognized by integrins on the cellular surface. Consequently, the adhesion of integrins to fibronectin is allowed and promotes fibronectin-fibril assembly, which implies that fibronectin is also a mechano-regulator of the extracellular matrix (Frantz et al. 2010; Pankov and Yamada 2002; Xu and Mosher 2011).
In contrast to the predominantly fibrillar structure of collagen and elastin, proteoglycans adopt highly extended conformations that are essential for hydrogel constitution (Mouw et al. 2014). The hydrogel molecules support the high compression forces in the extracellular matrix, around the cells and interstitial matrix. As stated above, the biological function of proteoglycans derives from the biochemical and hydrodynamic properties of the GAG macromolecules, which combine chemically with water to provide hydration and compression resistance. GAG has been classified into subtypes according to the function and structure of these carbohydrate chains, as well as the distribution, density and length of these chains concerning the core protein (Iozzo and Schaefer 2015). The most common GAG chains found in the ECM are heparin sulfate, chondroitin sulfate, dermatan sulfate, hyaluronan and keratin sulfate. The proteoglycans are encoded by a little less than 50 genes, besides several of them can be subject to alternative splicing, which shows the enormous variety of members of proteoglycans present in the matrix. They also have a vast range of functions such as cell adhesion, migration, proliferation, signaling, communication, morphogenesis and growth. In turn, proteoglycans also participate in angiogenesis, the inflammatory response to pathogens and injuries (Kresse and Schönherr 2001; Iozzo and Schaefer 2015). After having explained the unique features, functions and essential members of the extracellular matrix some techniques to replicate or mimic the extracellular matrix need to be shown.
Biomimetic Extracellular Matrix
The scaffolds designed to replicate the properties of the extracellular matrix must achieve several premises described above as morphology and mechanical properties. To set up a simple classification, they could be divided into two broads categories: organic scaffold materials and inorganic scaffold materials.
The inorganic scaffold materials can also be classified into different classes according to: (a) type and whole number of polymers constituent, (b) ability to be reabsorbed and replaced by the host tissue, (c) capability of chemical modification and bio-functionalization on their surface, (d) capability of covalent bond forming with some other materials (organic or inorganic), and finally, (e) type of manufacturing. The biodegradable polymers are preferred because they reduce the immune reaction to the foreign body, and also allow the recolonization of the damaged area allowing a more efficient regeneration. Polymers more frequently used for preparing inorganic scaffolds are poly(L-lactic acid) (PLLA), poly(glycolic acid) (PGA), poly(caprolactone) (PCL) and poly(lacticco-glycolic acid) (PLGA) (Hussey et al. 2018; Stratton et al. 2016).
The Organic Scaffold Materials (OSM), on the other hand, are those formed of macromolecules from a natural source from a living being, sometimes purified or treated as an extract. OSM also can include macromolecules as proteins over-expressed and purified in a cell host. Organic scaffold materials have several advantages, producing a low foreign body reaction, which is why, in general, they have a moderate inflammatory response. Besides, organic materials are biodegradable by enzymes that are naturally found in the body. Moreover, organic materials can easily be mixed with inorganic materials to form composite materials with new physical and chemical properties. Among them, biopolymers more frequently used for preparing natural scaffolds are collagen, fibronectin, glycosaminoglycans, fibrin, silk proteins, alginate, chitosan, hyaluronic acid and cellulose (Mano et al. 2007; Guarino et al. 2012).
Nanofibers
One of the goals of dental tissue engineering is to mimic the extracellular matrix; to achieve this goal, different technologies have been developed including Air-Jet Spinning (AJS) and electro spinning (ES). Several studies have demonstrated that AJS and ES can be successfully used to fabricate biomimetic analogs able to respond to the fibrillar architecture of the extracellular matrix (Braghirolli et al. 2014; Stojanovska et al. 2016; Guarino et al. 2016).
Air jet spinning (AJS) is a technique to produce spun microfibers and nanofibers of polymeric materials and composite materials. This technique is based on the use of air regulated pressure to apply as a jet or spray the polymer solution as thin fibers onto the surface and may also be passed or conducted under controlled pulsations directly through the nozzle. As the polymer solution leaves the AJS nozzle, the solvent evaporates during the formation and deposition of the fibers onto the surface. The system has many advantages such as: easy to use, not requiring sophisticated equipment, scalability, short period for manufacturing and low cost (Suarez-Franco et al. 2018; Lasprilla et al. 2012).
Electrospinning is a technique which uses the basic principles of AJS. However, the driving force to generate the fibers from a polymer solution consists of the high voltage electric field applied between a metallic nozzle and the grounded collector where the fibers will be deposited. Electrospinning shows an essential advantage related to the capability to reduce fiber size down to several nanometers in diameter, despite this some limitations still remain, such as low production rates and high dependency on polymer properties. However, this technique, i.e., AJS, is easy to use and with high adaptability to several conditions and different materials (Guarino et al. 2018; Sill and von Recum 2008). For these reasons, AJS and electrospinning have gained considerable attention in the past several years as technology for mimicking the fibrous structure and sizes of the extracellular matrix with several applications on oral and craniofacial tissue engineering. Due to the versatility, adaptability and easy handling of the fibers several studies were performed to increase the bioactivity and biofunctionality of fibers, by incorporating different dopants. Scaffold doping may be divided into two categories depending on whether this is non-covalent or covalent bonding between the fibers and the dopant (Ji et al. 2011). Non-covalent binding to the fibers could be guided by distinct forces as electronegativity, van der Waals forces, hydrogen bonds or the dopants could be adsorbed or wrapped by the polymer (Park and Ji 2011). The covalent bonding to the fibers is accomplished by chemical modification to produce a reactive functional group to immobilize a bioactive dopant to the polymer (Yoo et al. 2009). These processes can change the behavior of the fiber dramatically and improve the cellular response for healing.
Some non-covalent composite scaffolds are polylactic acid (PLA)-hydroxyapatite (HA), copolymer PLGA [PLA + polyglycolide (PGA)] -titania, poly (methyl methacrylate) (PMMA)-carbon nanotubes (CNTs) (Gloria et al. 2010; Luong et al. 2008). These composite scaffolds have different physical and chemical properties in comparison with the pure polymer scaffolds. PLA-HA shows a high surface free energy and low water contact angle, because this degradation rate was slow as compared with PLA scaffold. Also, fibers were doped with different drugs, and they even have added organic compounds such as proteins, peptides and growth factors to develop other types of non-covalent composite scaffolds. In a recent study using a syringe pump coupled to two gas-brush devices, demonstrated depositing PLGA fibers with proteins physisorbed and/or adsorbed as collagen and fibrin. This scaffold with deposited proteins shows capability of fibroblast migrations towards the fibers, arrangements of cells in similar conformations to the connective tissues and high expression of ECM proteins (Kaufman et al. 2018). However, several studies have demonstrated that surface structure, hydrophobicity and charge affect the protein structure when they are adsorbed to a solid-liquid interface. The most crucial step in protein absorption on the biomaterial surface is the release of water. In this way, the interface protein biomaterial surface is separated by two interfaces: on the one hand surface and water interface and the other hand protein and water interface. The process of protein adsorption over the surface could cause changes in the protein structure, which could induce the denaturalization of proteins (Ostuni et al. 2001). These structural changes on protein adsorption are due to the native folding of the protein that corresponds to the free energy minimum in solution, and does not compare to the free energy minimum once the protein is in contact with the surface (Rabe et al. 2011).
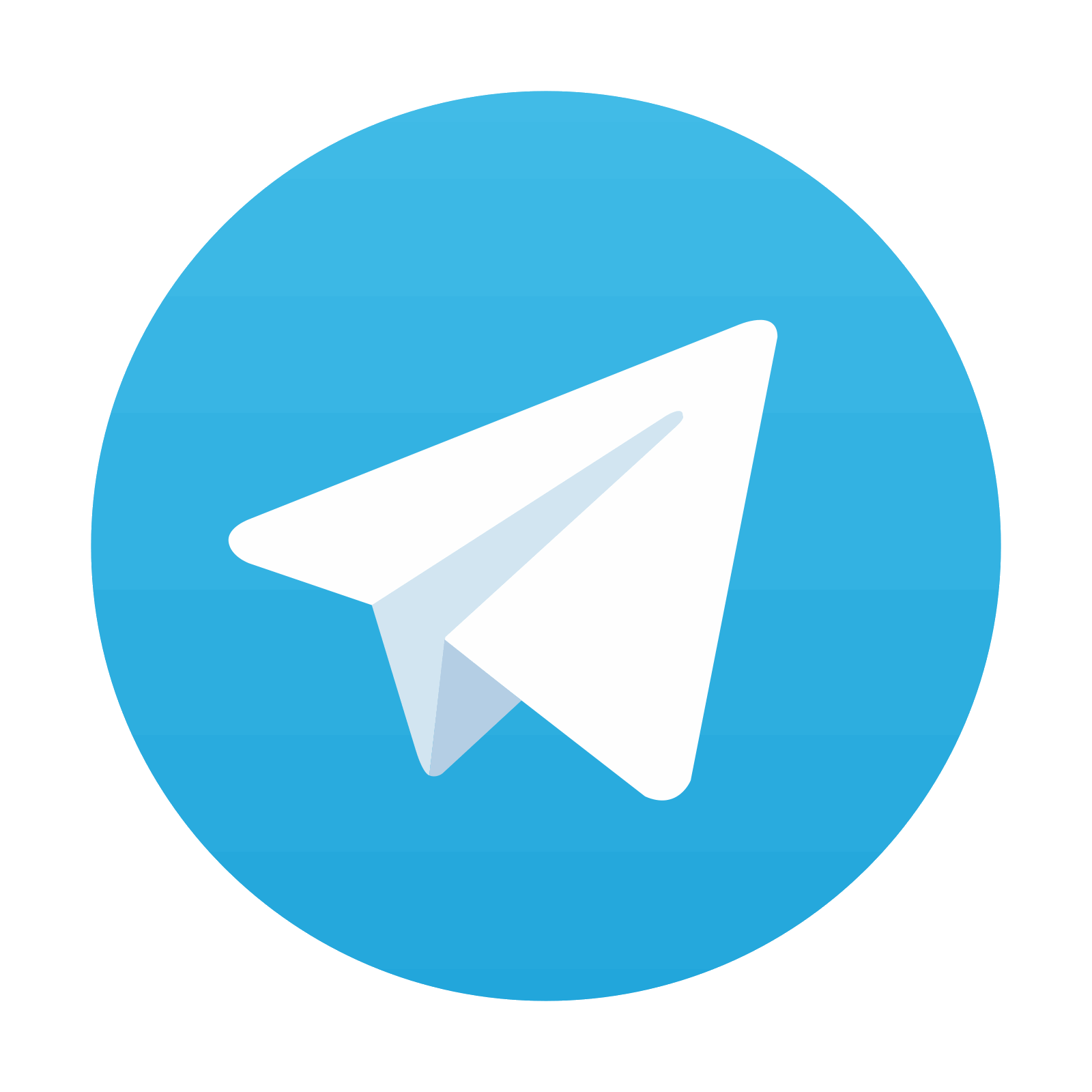
Stay updated, free dental videos. Join our Telegram channel

VIDEdental - Online dental courses
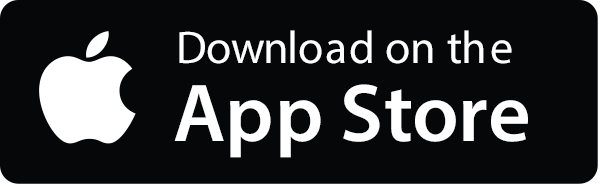
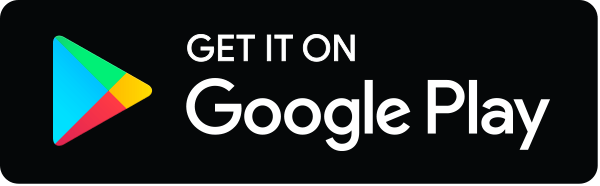