Graphical abstract

Highlights
- •
Relationship between self-healing capacity and initiator concentration.
- •
Relationship between self-healing capacity and microcapsule size and concentration.
- •
Implementation of microcapsule-based self-healing in commercial dental composites.
Abstract
Objective
Fracture is one of the main causes for failure of resin-based composite restorations. To overcome this drawback, self-healing resin-based composites have been designed by incorporation of microcapsules. However, the relationship between their self-healing capacity and microcapsule and resin parameters is still poorly understood. Therefore, the objective of this study was to systematically investigate the effect of initiator concentration (in the resin) and microcapsule size and concentration on the self-healing performance of commercially available flowable resin-based composites.
Methods
Poly(urea-formaldehyde) (PUF) microcapsules containing acrylic healing liquid were synthesized in small (33 ± 8 μm), medium (68 ± 21 μm) and large sizes (198 ± 43 μm) and characterized. Subsequently, these microcapsules were incorporated into a conventional flowable resin-based composite (Majesty Flow ES2, Kuraray) at different contents (5–15 wt%) and benzoyl peroxide (BPO) initiator concentrations (0.5–2.0 wt%). Fracture toughness ( K IC ) of test specimens was tested using a single edge V-notched beam method. Immediately after complete fracture ( K IC-initial ), the two fractured parts were held together for 72 h to allow for healing. Subsequently, fracture toughness of the healed resin-based composites ( K IC-healed ) was tested as well.
Results
The fracture toughness of healed dental composites significantly increased with increasing microcapsule size and concentration (2 wt% BPO, p < 0.05). The highest self-healing efficiencies (up to 76%) were obtained with microcapsules sized 198 ± 43 um.
Significance
commercially available resin-based composites can be rendered self-healing most efficiently by incorporation of large microcapsules (198 ± 43 μm). However, long-term tests on fatigue and wear behavior are needed to confirm the clinical efficacy.
1
Introduction
Resin-based dental composites are increasingly popular in clinical practice due to their esthetics and improved physical properties . However, microcracks can be formed in such composites due to repeated mechanical loading in the oral environment . The evolution of these microcracks can eventually lead to material fracture, which is one of the main causes for premature failure of resin-based composite restorations . To improve the long-term mechanical performance of dental composites, self-healing resin-based composites have been developed by Xu et al. , which are inspired by the initial concept for extrinsic self-healing proposed by White et al. . These smart dental materials exhibit a unique capacity for self-healing by filling microcracks with polymerizable healing liquids before further crack propagation . Since then, several studies have been published on self-healing resin-based composites , which have been recently reviewed by Althaqafi et al. .
Extrinsic self-healing dental composites typically rely on microfracture-induced interaction between microcapsules containing a polymerizable healing liquid (consisting of acrylic monomers of the resin matrix) and initiators in the matrix which trigger the polymerization of this acrylic healing liquid. The self-healing process itself can be divided into several stages . After initial formation of microcracks, these cracks will grow until neighboring microcapsules rupture and release their healing liquid into the growing cracks. The extent of microcapsule rupturing depends on the relationship between the mechanical strength of the microcapsules and the forces generated within the composite. Subsequently, the polymerizable healing liquid can flow into the fracture plane or microcracks through capillary action. Polymerization of the healing liquid will be initiated by contact between the monomers of the acrylic healing liquid and initiator molecules embedded in the matrix. Complete polymerization of the healing liquid will stop further crack propagation.
Several studies have been reported on the influence of microcapsule properties on the extrinsic self-healing capacity of fully polymeric matrices such as poly(methyl methacrylate) (PMMA) . However, the influence of relevant microcapsule parameters such as microcapsule size on the self-healing capacity of glass filler-reinforced dental composites is still poorly understood. Moreover, the concept of extrinsic self-healing (containing monomer healing liquid) has only been confirmed in experimental systems but not yet successfully implemented in commercially available dental resin-based composite formulations.
Therefore, this study aimed to systematically investigate the effect of microcapsule size on the extrinsic self-healing capacity of commercially available flowable resin-based composites. Additionally, the influence of initiator concentration and microcapsule concentration on the self-healing capacity of these composite formulations was tested as well. We hypothesized that (1) the self-healing capacity of the resin-based composites would increase with increasing initiator concentration, microcapsule size and microcapsule concentration, and (2) commercially available resin-based composites could be rendered self-healing by incorporation of microcapsules.
2
Materials and methods
2.1
Preparation of microcapsules
Poly(urea-formaldehyde) (PUF) microcapsules containing an acrylic healing liquid were synthesized using an oil-in-water method . Urea (Sigma, UK) and formaldehyde solution (37 wt%, Sigma, Switzerland) were used to form the microcapsule shell. The healing liquid was composed of triethylene glycol dimethacrylate (TEGDMA, Sigma–Aldrich, Switzerland) and N,N-Dimethyl-p-toluidine (DEPT, Merck KGaA, India) (“oil phase”). 2.5 g ethylene-maleic anhydride (EMA, Sigma, UK) was fully dissolved into Milli-Q water (97.5 g) to prepare the aqueous solution. The “water phase” was prepared by adding 26 mL EMA solution to 100 ml Milli-Q water at room temperature. Subsequently, a lab mixer (IKA Works (Asia), RW20 DZM. N, Sdn. Bhd. Malaysia) with a three-bladed propeller (radius: 20 mm) was used to stir the solution at 300 rpm . Subsequently, 2.5 g urea, 0.25 g ammonium chloride (Merck KGaA, Germany) and 0.25 g resorcinol (Sigma–Aldrich, India) were added to this aqueous solution. Ammonium chloride and resorcinol were added with the aim to improve the strength and toughness of the microcapsules . In order to achieve microcapsules of large, medium and small size, the stirring speed was varied from 400 rpm (large size) to 800 rpm (medium size) and 1200 rpm (small size), respectively . Lower stirring speeds resulted in excessively large microcapsules, stable emulsion droplets could not be formed at higher stirring speeds. The pH was adjusted to 3.5 by addition of 1 M sodium hydroxide solution to the solution. Subsequently, 40 mL self-healing liquid (mixture of TEGDMA 99 wt% and DEPT 1 wt%) was added dropwise to the solution using a single syringe infusion pump (KDS, Infusion), after which 6.3 g formaldehyde solution was added to this emulsion in order to initiate the formation of the microcapsule shells. Thereafter, the emulsion was placed in a water-bath at 55 °C for 4 h. After polymerization of the microcapsule shells, the emulsion was cooled down and the microcapsules were filtered using a vacuum filter. Finally, the microcapsules were washed with water and acetone and were air-dried at room temperature for 24 h.
2.2
Characterization of microcapsules
Scanning electronic microscopy (SEM, Zeiss, Gemini) was used to characterize the morphology of the microcapsules. Based on the SEM images, the microcapsule size and shell thickness were measured (Imaging software Fiji, Image J 1.47v). Microcapsule size was measured by counting 150-200 microcapsules, while measurement of shell thickness was based on counting 10 shell images.
Fourier-transform infrared spectroscopy (FTIR, PerkinElmer, Spectrum Two) was applied to confirm successful encapsulation of the healing liquid in the microcapsules. The amount of encapsulated healing liquid was tested by thermogravimetric analysis (TGA, Mettler Toledo, TAG/DSC2) and an acetone washing-based method . For the TGA test, microcapsule samples were heated from 20 °C to 500 °C with a heating rate of 10 °C min −1 . For the acetone washing method, a known amount of microcapsules was squashed and washed using acetone to remove the self-healing liquid. The remaining microcapsule shells were weighted to calculate the amount of healing liquid in the samples.
2.3
Preparation of self-healing composites
To prepare the microcapsule-enriched self-healing resin-based composites, we manually mixed the microcapsules, initiator (benzoyl peroxide, BPO, ACROS, New Jersey, USA) and the commercially available flowable composite (Clearfil Majesty™ ES Flow, Kuraray, Japan) and applied the mixture into rectangular molds (polydimethylsiloxane, PDMS) in size 12.5 mm × 5 mm × 2.5 mm. Various groups with different concentrations of initiator (0.5 wt%, 1.0 wt%, 2.0 wt%), microcapsules (5 wt%, 10 wt% and 15 wt%) and microcapsule size (small, medium and large) were prepared (see Table 1 for composition of various experimental groups). Composites were cured for 20 s (both sides) with a LED polymerization unit (Bluephase 16i, Ivoclar Vivadent, output >1300 mW/cm 2 ). SEM analysis was performed on the healed fracture surfaces, which were fractured by a universal testing machine (AMETEK, LLOYD, LS5) and tightly put back together with rubber bands (Ormco Corporation, Mexico) for 72 h for healing at room temperature (∼21 °C).
Test | Group | Initiator concentration (wt%) | Microcapsule size | Microcapsule concentration (wt%) |
---|---|---|---|---|
Effect of initiator concentration on self-healing performance | Control | 0 | – | 0 |
1 | 0.5 | Large | 10 | |
2 | 1.0 | Large | 10 | |
3 | 2.0 | Large | 10 | |
Effect of microcapsule size and concentration on self-healing performance | Control | 0 | – | 0 |
1 | 2.0 | Large | 5 | |
2 | 2.0 | Medium | 5 | |
3 | 2.0 | Small | 5 | |
4 | 2.0 | Large | 10 | |
5 | 2.0 | Medium | 10 | |
6 | 2.0 | Small | 10 | |
7 | 2.0 | Large | 15 | |
8 | 2.0 | Medium | 15 | |
9 | 2.0 | Small | 15 |
2.4
Fracture toughness and self-healing efficiency
Fracture toughness was tested by the single-edge V-notched method. In each sample, a notch with a depth of 1/3 thickness and width 0.7 mm, was prepared at the center of one side with a customized cutting machine. Then, a pre-crack was produced by sliding a sharp razor blade (10 times) in the bottom of the notch. All samples ( n = 8 per experimental group) were stored at room temperature in deionized water for 24 h before testing and were subjected to three-point bending tests using a universal testing machine (AMETEK, LLOYD, LS5). Fracture toughness K IC was calculated with the formulas below .
KIC=PLbw1.5faw
wheref(aw)=3α(aw)0.5{1.99−(aw)(1−aw)[2.15−3.93aw+2.7(aw)2]}
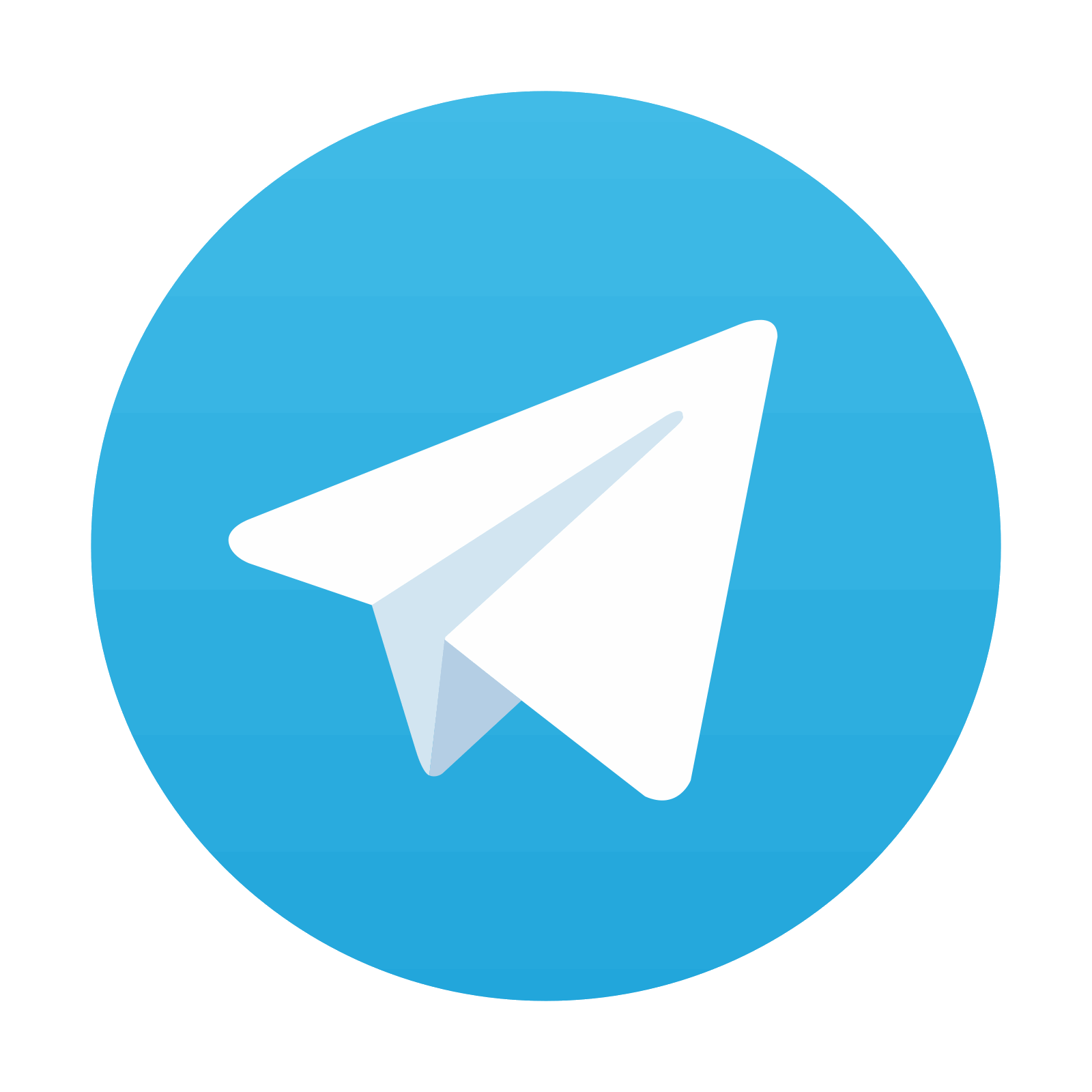
Stay updated, free dental videos. Join our Telegram channel

VIDEdental - Online dental courses
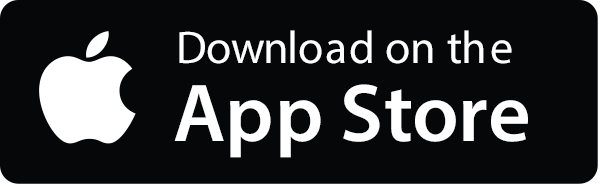
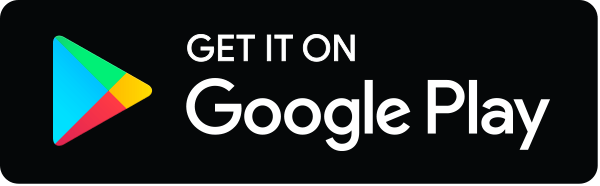
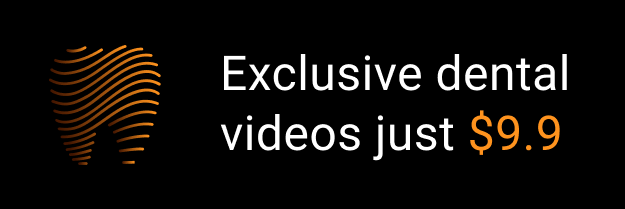