Highlights
- •
Electrical properties of remineralizing composite materials were investigated.
- •
Polymerization kinetics were assessed by changes in electrical resistivity.
- •
Resistivity values correlated well with degree of conversion.
- •
Polymerization reaction rate was affected by light transmittance.
- •
Electrical resistivity was affected by water content introduced by filler particles.
Abstract
Objectives
To investigate the electrical properties of experimental light-curable composite materials based on amorphous calcium phosphate (ACP) with the admixture of silanized barium glass and silica fillers.
Methods
Short-term setting was investigated by impedance measurements at a frequency of 1 kHz, while for the long-term setting the impedance spectra were measured consecutively over a frequency range of 0.05 Hz to 1 MHz for 24 h. The analysis of electrical resistivity changes during curing allowed the extraction of relevant kinetic parameters. The impedance results were correlated to the degree of conversion assessed by Raman spectroscopy, water content determined by gravimetry, light transmittance measured by CCD spectrometer and microstructural features observed by scanning electron microscopy.
Results
ACP-based composites have shown higher immediate degree of conversion and less post-cure polymerization than the control composites, but lower polymerization rate. The polymerization rate assessed by impedance measurements correlated well with the light transmittance. The differences in the electrical conductivity values observed among the materials were correlated to the amount of water introduced into composites by the ACP filler. High correlation was found between the degree of conversion and electrical resistivity. Equivalent circuit modeling revealed two electrical contributions for the ACP-based composites and a single contribution for the control composites.
Significance
The impedance spectroscopy has proven a valuable method for gaining insight into various features of ACP-based composites. Better understanding of the properties of ACP-based composites should further the development of these promising bioactive materials.
1
Introduction
Incorporation of amorphous calcium phosphate (ACP) fillers in methacrylate resins results in bioactive, ion-releasing composite materials. Their bioactivity is due to the thermodynamic instability of ACP, which in aqueous media spontaneously converts into hydroxyapatite and concomitantly releases calcium and phosphate ions . When embedded in the appropriate methacrylate resin, ACP provides a sustained release of calcium and phosphate ions in concentrations sufficient for hydroxyapatite precipitation in dental hard tissues adjacent to the restoration . The remineralizing ability of ACP-based composites has been demonstrated in vitro , in situ and in animal model in vivo . These findings indicate the potential of ACP-based composites for preventing secondary caries, which is considered the main cause for failure of contemporary composite restorations .
The major drawbacks of ACP-based composites are poor mechanical properties and high shrinkage stress in comparison to commercial composites . To address these issues, ACP-based formulations were introduced with conventional silanized fillers . Since the ACP-based composites attain sufficient ion release with 40 wt% of ACP , their filler load can be increased by the addition of inert fillers similar to those contained in conventional composites. In this way, a “hybrid” material is composed, which consists of a bioactive part (ACP fillers) and a reinforcing part (inert fillers). When exposed to water, ACP undergoes a dissolution-reprecipitation process converting into hydroxyapatite and releasing ions , while the inert fillers remain bonded to the methacrylate network and reinforce the restoration. The admixture of inert fillers (barium glass and silica) has been shown to improve the mechanical properties, without compromising the ion release . By fine-tuning the composition of such “hybrid” ACP-based composites, their flexural strength may be enhanced to match the values of commercial resin-modified glass ionomer cements and possibly even commercial composites .
Various properties of ACP-based composites have been investigated, but there are no studies examining their electrical properties by means of impedance spectroscopy (IS). Generally, IS is rarely used in investigations of dental composites—only several papers are available, dealing with composite degradation , water sorption , microleakage and polymerization kinetics . In particular, Steinhaus et al. have described the kinetics of composite polymerization from the changes of the electrical resistivity as a function of time, ρ ( t ), using the relation: ρ ( t ) = ρ init + B × Δ t ; where ρ init is the initial resistivity measured before the curing and Δ t is the time period since the start of curing. In the linear range of the resistivity vs. time curve, the resistivity is assumed to be proportional to the rheological viscosity and molecular mass of the growing polymeric chains. Therefore, slope B can be used as a measure of reaction rate during an early stage of polymerization, while polymeric chains grow linearly. Another kinetic parameter in the model is the reaction time constant, τ , contained in the relation: Δ ρ ( t ) = Δ ρ max × e −(Δ t / τ ) where Δ ρ ( t ) is the change of resistivity as a function of time and Δ ρ max is the total change of resistivity during curing.
All above-mentioned IS studies are performed at a fixed frequency and there are no reports of the electrical properties of dental composites measured in a wide frequency range. This study employs IS to investigate the structural changes occurring during the short-term setting (during light-curing) and long-term setting (up to 24 h post-cure) of five ACP-based and two control composites. The short-term setting is analyzed in terms of impedance (electrical conductivity) changes at a fixed frequency as a function of time, while for the long-term setting the impedance spectra were measured consecutively over a frequency range of 0.05 Hz to 1 MHz for 24 h. Furthermore, the results obtained by IS are correlated to the degree of conversion, water content, light transmittance and microstructural features.
2
Materials and methods
2.1
Composite materials
The composition of composite materials is given in Table 1 . The synthesis of zirconia-hybridized ACP (Zr-ACP) fillers followed the procedure by Skrtic et al. . The resin was blended with fillers in lightproof containers using a dual asymmetric centrifugal mixing system (Speed Mixer TM DAC 150 FVZ, Hauschild & Co. KG, Hamm, Germany), followed by deaerating the obtained composite pastes in vacuum for 12 h.
Material | Filler (wt%) | Resin (wt%) | Filler load (vol%) | ||
---|---|---|---|---|---|
ACP-based materials | ACP40 | 40% Zr-ACP | 60 wt% | 67% EBPADMA | 27.6 |
ACP40-Ba10 | 40% Zr-ACP, 10% Ba-fillers | 50 wt% | 23% TEGDMA | 35.1 | |
ACP40-Si10 | 40% Zr-ACP, 10% Si-fillers | 50 wt% | 10% HEMA | 36.6 | |
ACP40-Ba5Si5 | 40% Zr-ACP, 5% Ba-fillers, 5% Si-fillers | 50 wt% | Photoinitiator: | 35.9 | |
ACP40- Ba9Si1 | 40% Zr-ACP, 9% Ba-fillers, 1% Si-fillers | 50 wt% | 0.2% CQ | 35.3 | |
control materials | Ba40 | 40% Ba-fillers | 60 wt% | 0.8% 4E | 22.0 |
Ba40Si10 | 40% Ba-fillers, 10% Si-fillers | 50 wt% | 31.5 |
2.2
Impedance spectroscopy
The samples ( n = 3) were cast in quartz rings ( d = 4 mm, h = 4 mm), covered by brass electrodes ( d = 4 mm) and placed in an impedance cell. Complex impedance was measured using an impedance analyzer (Novocontrol Alpha-AN Dielectric Spectrometer, Novocontrol Technologies GmbH & Co. KG, Germany) at 2 V. Measurements at the fixed frequency of 1 kHz and consecutive frequency sweeps from 0.05 Hz to 1 MHz were performed to monitor the short-term and long-term setting, respectively. Frequency sweeps were performed every 5 min during the first 3 h and afterwards at 6 h, 12 h and 24 h.
For light curing of the samples, LED polymerization devices were used (Bluephase G2, Ivoclar-Vivadent, Schaan, Liechtenstein; wavelength range 380–515 nm, intensity 1200 mW/cm 2 ). The light probes of two polymerization devices were placed immediately adjacent to the quartz rings and parallel to the brass electrodes, diametrically opposite to one another. To assure a thorough curing throughout the sample, the first polymerization device was activated for 30 s, followed by a successive activation of the second one for additional 30 s.
From complex impedance ( Z *), the electrical conductivity ( σ *) was calculated using the relation σ * = 1/ Z * × h / A where h is the height and A is the cross-sectional area of the sample. The short-term setting results were presented as changes of the real part of conductivity, σ ’, and resistivity ( ρ * = 1/ σ *) as a function of time. The linear part of the resistivity curve was fitted to a straight line ( R 2 > 0.99), and curing kinetics were assessed using parameters slope and τ , as described previously by Steinhaus et al. . The impedance spectra were analyzed by means of equivalent circuit modeling and parameters were obtained using the complex non-linear least square (CNLLSQ) fitting using ZView software.
2.3
Gravimetric analysis
For each material, six discs ( d = 5 mm, h = 2 mm) were polymerized using the Bluephase device for 40 seconds on each side. The samples were weighted to the accuracy of 10 −4 g with the analytical balance (XS204, Mettler Toledo, Greifensee, Switzerland) immediately after polymerization, after 5 days and 14 days of desiccator storage. The equilibrium occurred after 5 days, thus the water content (wt%) was calculated as: ((mass before drying) − (mass after drying))/(mass before drying).
2.4
Light transmittance
Uncured composite materials were cast into cylindrical Teflon molds ( d = 6 mm, h = 2 mm), covered from both sides with a polyethylene terephthalate (PET) film (0.05 mm thick) and sandwiched between two glass plates ( h = 1 mm). The curing unit tip was positioned immediately below the glass plate and the spectra were taken from the opposite side of the sample by a CCD array fiber spectrometer HR4000 (Ocean Optics, Dunedin, FL, USA). Transmittance was recorded at the wavelength of 468 nm immediately after activation of the curing unit and five repeats were made for each composite. The ratio of the light intensity passing through the sample and the light intensity passing through the empty sample compartment was calculated as the transmittance (%).
2.5
Scanning electron microscopy (SEM)
The light-cured (40 s), disc-shaped samples of 1 mm thickness, unpolished and uncoated were examined with FE-SEM JSM 7000 (JEOL, Peabody, MA, USA).
2.6
Raman spectroscopy
Five cylindrical samples ( d = 3 mm, h = 2 mm) were made using a stainless steel mold. Uncured composite was cast into the mold, the mold apertures were covered with a PET film and curing was performed for 40 s through the upper aperture with the Bluephase device. The curing unit tip was positioned at the angle of 90°, immediately adjacent to the mold aperture, contacting the PET film covering the sample. Raman spectra were recorded from the upper sample surface immediately after curing and after 24 h of dark storage in the incubator (Cultura, Ivoclar-Vivadent, Schaan, Liechtenstein) at 37 ± 1 °C.
FT-Raman spectroscopy measurements were performed using a Spectrum GX spectrometer (PerkinElmer, Waltham, USA). The excitation was an NdYaG laser at 1064 nm wavelength, with laser power of 400 mW and resolution of 4 cm −1 . The exposed sample surface was 0.5 mm in diameter. For each spectrum, 100 scans were recorded. Spectra of the uncured composites ( n = 5) were recorded in the same manner. The spectra were processed with the Kinetics add-on for Matlab (Mathworks, Natick, Massachusetts, USA).
Degree of conversion calculation was performed by comparing the relative change of the band at 1640 cm −1 , representing the aliphatic C C stretching mode to the aromatic C C band at 1610 cm −1 , before and after polymerization. Peak heights of aliphatic C C and aromatic C C bands were used for the conversion calculation by the following equation: conversion = 1 − R polymerized / R unpolymerized , where R = (aliphatic C C peak height)/(aromatic C C peak height) .
It is important to note that the sample geometries differed among the methods due to technical reasons. The curing regimes used for each of the sample geometries were adapted to attain the maximum conversion throughout the bulk of the samples, as confirmed by preliminary measurements. Thus the samples were assumed to be fully and homogeneously polymerized, allowing comparison of the results obtained from different setups. For all the experiments, sample preparation and measurements were done at room temperature of 21 ± 1 °C.
2.7
Statistical analysis
Descriptive statistics were used to present the data and Pearson’s correlation analysis was used to correlate the water content, conversion, filler load and transmittance with impedance data ( α = 0.05). One-way ANOVA with post-hoc Tukey test was used for comparison of conversion among the composites, while the repeated samples t -test was used for comparison of conversion immediately after curing and 24 h post-cure ( α = 0.05). Statistical analysis was performed in SPSS 20 (IBM, Armonk, NY, USA)
2
Materials and methods
2.1
Composite materials
The composition of composite materials is given in Table 1 . The synthesis of zirconia-hybridized ACP (Zr-ACP) fillers followed the procedure by Skrtic et al. . The resin was blended with fillers in lightproof containers using a dual asymmetric centrifugal mixing system (Speed Mixer TM DAC 150 FVZ, Hauschild & Co. KG, Hamm, Germany), followed by deaerating the obtained composite pastes in vacuum for 12 h.
Material | Filler (wt%) | Resin (wt%) | Filler load (vol%) | ||
---|---|---|---|---|---|
ACP-based materials | ACP40 | 40% Zr-ACP | 60 wt% | 67% EBPADMA | 27.6 |
ACP40-Ba10 | 40% Zr-ACP, 10% Ba-fillers | 50 wt% | 23% TEGDMA | 35.1 | |
ACP40-Si10 | 40% Zr-ACP, 10% Si-fillers | 50 wt% | 10% HEMA | 36.6 | |
ACP40-Ba5Si5 | 40% Zr-ACP, 5% Ba-fillers, 5% Si-fillers | 50 wt% | Photoinitiator: | 35.9 | |
ACP40- Ba9Si1 | 40% Zr-ACP, 9% Ba-fillers, 1% Si-fillers | 50 wt% | 0.2% CQ | 35.3 | |
control materials | Ba40 | 40% Ba-fillers | 60 wt% | 0.8% 4E | 22.0 |
Ba40Si10 | 40% Ba-fillers, 10% Si-fillers | 50 wt% | 31.5 |
2.2
Impedance spectroscopy
The samples ( n = 3) were cast in quartz rings ( d = 4 mm, h = 4 mm), covered by brass electrodes ( d = 4 mm) and placed in an impedance cell. Complex impedance was measured using an impedance analyzer (Novocontrol Alpha-AN Dielectric Spectrometer, Novocontrol Technologies GmbH & Co. KG, Germany) at 2 V. Measurements at the fixed frequency of 1 kHz and consecutive frequency sweeps from 0.05 Hz to 1 MHz were performed to monitor the short-term and long-term setting, respectively. Frequency sweeps were performed every 5 min during the first 3 h and afterwards at 6 h, 12 h and 24 h.
For light curing of the samples, LED polymerization devices were used (Bluephase G2, Ivoclar-Vivadent, Schaan, Liechtenstein; wavelength range 380–515 nm, intensity 1200 mW/cm 2 ). The light probes of two polymerization devices were placed immediately adjacent to the quartz rings and parallel to the brass electrodes, diametrically opposite to one another. To assure a thorough curing throughout the sample, the first polymerization device was activated for 30 s, followed by a successive activation of the second one for additional 30 s.
From complex impedance ( Z *), the electrical conductivity ( σ *) was calculated using the relation σ * = 1/ Z * × h / A where h is the height and A is the cross-sectional area of the sample. The short-term setting results were presented as changes of the real part of conductivity, σ ’, and resistivity ( ρ * = 1/ σ *) as a function of time. The linear part of the resistivity curve was fitted to a straight line ( R 2 > 0.99), and curing kinetics were assessed using parameters slope and τ , as described previously by Steinhaus et al. . The impedance spectra were analyzed by means of equivalent circuit modeling and parameters were obtained using the complex non-linear least square (CNLLSQ) fitting using ZView software.
2.3
Gravimetric analysis
For each material, six discs ( d = 5 mm, h = 2 mm) were polymerized using the Bluephase device for 40 seconds on each side. The samples were weighted to the accuracy of 10 −4 g with the analytical balance (XS204, Mettler Toledo, Greifensee, Switzerland) immediately after polymerization, after 5 days and 14 days of desiccator storage. The equilibrium occurred after 5 days, thus the water content (wt%) was calculated as: ((mass before drying) − (mass after drying))/(mass before drying).
2.4
Light transmittance
Uncured composite materials were cast into cylindrical Teflon molds ( d = 6 mm, h = 2 mm), covered from both sides with a polyethylene terephthalate (PET) film (0.05 mm thick) and sandwiched between two glass plates ( h = 1 mm). The curing unit tip was positioned immediately below the glass plate and the spectra were taken from the opposite side of the sample by a CCD array fiber spectrometer HR4000 (Ocean Optics, Dunedin, FL, USA). Transmittance was recorded at the wavelength of 468 nm immediately after activation of the curing unit and five repeats were made for each composite. The ratio of the light intensity passing through the sample and the light intensity passing through the empty sample compartment was calculated as the transmittance (%).
2.5
Scanning electron microscopy (SEM)
The light-cured (40 s), disc-shaped samples of 1 mm thickness, unpolished and uncoated were examined with FE-SEM JSM 7000 (JEOL, Peabody, MA, USA).
2.6
Raman spectroscopy
Five cylindrical samples ( d = 3 mm, h = 2 mm) were made using a stainless steel mold. Uncured composite was cast into the mold, the mold apertures were covered with a PET film and curing was performed for 40 s through the upper aperture with the Bluephase device. The curing unit tip was positioned at the angle of 90°, immediately adjacent to the mold aperture, contacting the PET film covering the sample. Raman spectra were recorded from the upper sample surface immediately after curing and after 24 h of dark storage in the incubator (Cultura, Ivoclar-Vivadent, Schaan, Liechtenstein) at 37 ± 1 °C.
FT-Raman spectroscopy measurements were performed using a Spectrum GX spectrometer (PerkinElmer, Waltham, USA). The excitation was an NdYaG laser at 1064 nm wavelength, with laser power of 400 mW and resolution of 4 cm −1 . The exposed sample surface was 0.5 mm in diameter. For each spectrum, 100 scans were recorded. Spectra of the uncured composites ( n = 5) were recorded in the same manner. The spectra were processed with the Kinetics add-on for Matlab (Mathworks, Natick, Massachusetts, USA).
Degree of conversion calculation was performed by comparing the relative change of the band at 1640 cm −1 , representing the aliphatic C C stretching mode to the aromatic C C band at 1610 cm −1 , before and after polymerization. Peak heights of aliphatic C C and aromatic C C bands were used for the conversion calculation by the following equation: conversion = 1 − R polymerized / R unpolymerized , where R = (aliphatic C C peak height)/(aromatic C C peak height) .
It is important to note that the sample geometries differed among the methods due to technical reasons. The curing regimes used for each of the sample geometries were adapted to attain the maximum conversion throughout the bulk of the samples, as confirmed by preliminary measurements. Thus the samples were assumed to be fully and homogeneously polymerized, allowing comparison of the results obtained from different setups. For all the experiments, sample preparation and measurements were done at room temperature of 21 ± 1 °C.
2.7
Statistical analysis
Descriptive statistics were used to present the data and Pearson’s correlation analysis was used to correlate the water content, conversion, filler load and transmittance with impedance data ( α = 0.05). One-way ANOVA with post-hoc Tukey test was used for comparison of conversion among the composites, while the repeated samples t -test was used for comparison of conversion immediately after curing and 24 h post-cure ( α = 0.05). Statistical analysis was performed in SPSS 20 (IBM, Armonk, NY, USA)
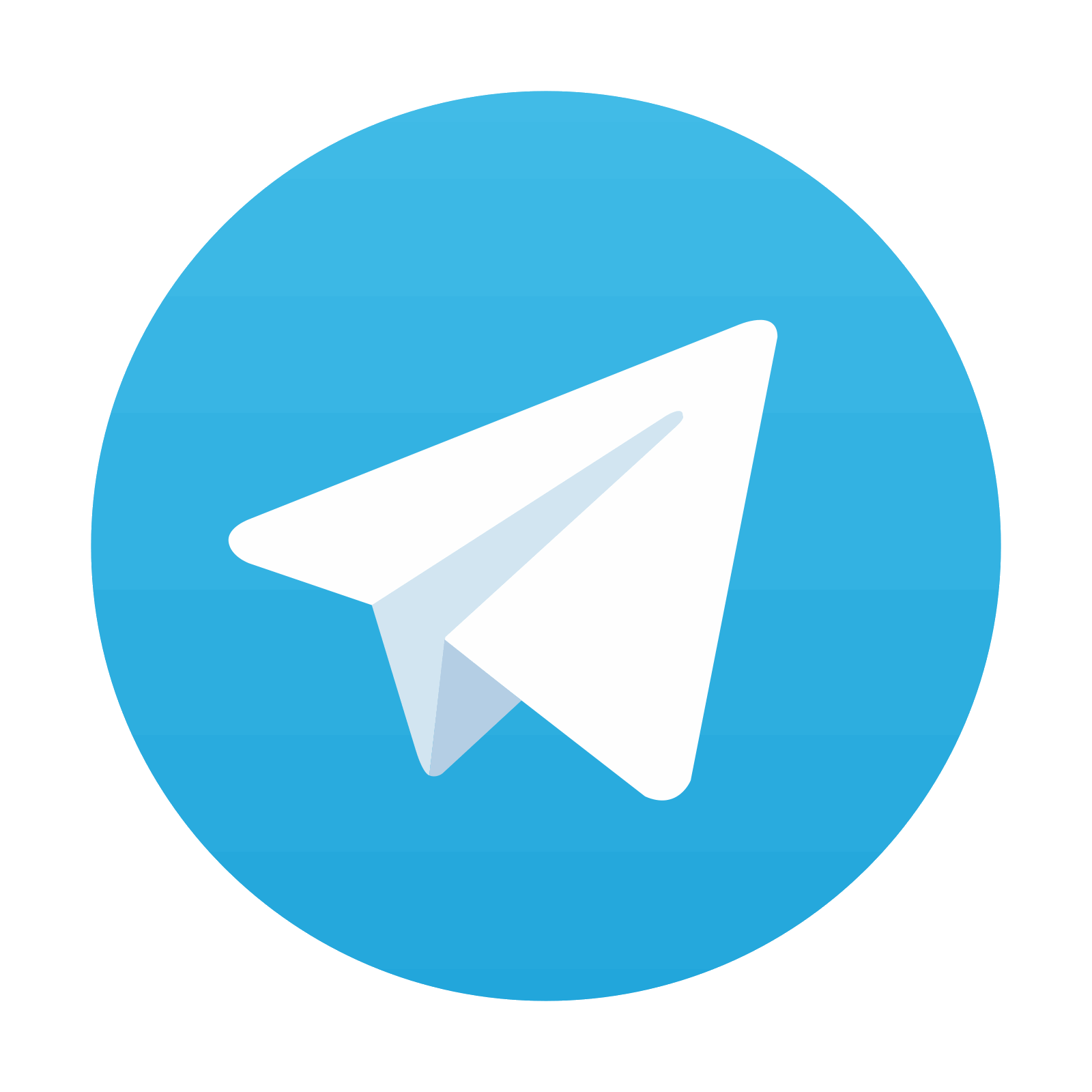
Stay updated, free dental videos. Join our Telegram channel

VIDEdental - Online dental courses
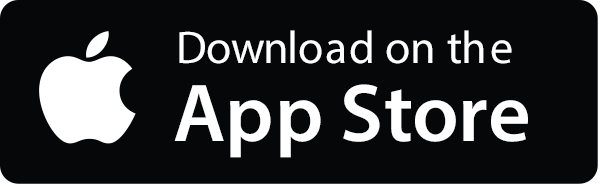
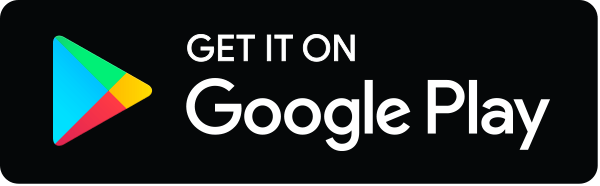