Periodontal disease is a major public health issue and the development of effective therapies to treat the disease and regenerate periodontal tissue is an important goal of today’s medicine. This article highlights recent scientific advancements in gene therapy, stem cell biology, and RNA interference with the intent of identifying their potential in periodontal tissue regeneration. Results from basic research, preclinical, and clinical studies indicate that these fields of research may soon contribute to more effective regenerative therapies for periodontal disease.
Periodontal disease is characterized by inflammation that leads to alveolar bone loss and destruction of periodontal ligament. Epidemiologic research shows that approximately 50% of the adult population between the ages of 45 and 65 years have periodontal disease. Recent scientific evidence highlights the connection between periodontitis and the pathogenesis or maintenance of other diseases. Periodontal disease is a public health issue and the development of effective therapies to treat periodontal disease and regenerate periodontal tissue is a major goal of the scientific community.
The challenges in regenerative periodontal therapy lie in the ability to induce the regeneration of a complex apparatus composed of different tissues, such as bone, cementum, and periodontal ligament. Despite the recent advancements in periodontal therapy, a complete regeneration of the damaged periodontium is still unattainable. Various therapeutic approaches, including the use of recombinant growth factors, have been applied and none of them have been shown to induce complete regeneration of the lost periodontium.
The regeneration of the periodontal tissue is no different in principle from the regeneration of any other body tissue. Currently, there are five main types of therapeutics used or in development for tissue regeneration: (1) conductive therapeutics, (2) inductive therapeutics, (3) cell-based therapeutics, (4) gene-based therapeutics, and (5) RNA-based therapeutics.
A conductive therapeutic is a biocompatible scaffold that guides the regeneration of the tissue by passively allowing the attachment and growth of vascular elements and progenitor stem cells that reside in the tissue defect. Its regenerative potential is limited by the lack of biologically active factors and sufficient progenitor cells within the defect. Examples of conductive therapeutics for periodontal regeneration are hydroxyapatite, tricalcium phosphate, and calcium sulfate fillers.
An inductive therapeutic is a biocompatible scaffold that guides and induces the regeneration of the tissue by carrying one or more biologically active factors that recruit vascular elements and progenitor stem cells from the immediate vicinity to the tissue defect. Its regenerative potential is higher than the conductive biomaterial because more progenitor cells can repopulate the tissue defect. Examples of inductive therapeutics for periodontal regeneration are allogenic bone grafts or biomaterials carrying osteoinductive recombinant proteins.
A cell-based therapeutic is a biocompatible scaffold that contains progenitor stem cells or differentiated cells. The cells are delivered within the tissue defect and become tissue-forming cells. Bone autografts may be considered as cell-based therapeutics for periodontal regeneration. As the result of stem cell research, cell-based therapeutics has been proposed for the delivery of undifferentiated or partially differentiated progenitor cells. Theoretically, the regenerative potential of a stem cell–based therapy is the highest, but limited by the scarce availability of sources for cells.
A gene-based therapeutic is a biocompatible scaffold carrying single or multiple genes that transform the nonprogenitor cells already present within the tissue defect into both progenitor and mature tissue-specific cells. As such, a gene therapy product does not need to carry and deliver progenitor cells within the tissue defect. Rather, it is able to signal the cells present in the defect to differentiate into a phenotype more favorable to the regeneration process, and it represents an attractive solution for regenerative therapy.
Because of their enormous potential, RNA-based therapeutics may be considered a fifth category of regeneration therapy even though this approach remains in a conceptual stage of development. They are based on the principle of RNA interference (RNAi), a novel mechanism of action by which RNAs silence gene expression. Novel therapeutic approaches may be envisioned whereby the expression of certain genes detrimental to the tissue regeneration process is silenced by RNAs.
This article analyzes the advances made by the scientific community in terms of periodontal regeneration, mainly focusing on gene-based and stem cell–based therapeutics. Additionally, the potential for RNAi as a new tool in modern regenerative biology is discussed.
Gene therapy
The Biology of Gene Therapy
The gene therapy approach to tissue regeneration presents the advantage of potentially mimicking the complex natural process of tissue formation. One or more genes encoding for growth factors or transcriptional regulators may be delivered within the tissue defect, fostering the regeneration of the missing tissue. These factors may be delivered simultaneously or sequentially to control the timing and distribution of the delivered factors, resembling their expression during the healing process.
Most studies of gene therapy for tissue regeneration have been conducted using either adenovirus vectors, adenoassociated viruses, retroviruses, lentiviruses, or naked DNA. Adenoviruses have the advantage of not depending on cell replication for the delivery of DNA. In addition, adenoviral DNA does not normally integrate into the host genome. For this reason, the transgene expression from adenoviruses is quite brief (up to 2 weeks according to some recent research). Cells infected by adenoviruses may secrete viral proteins, however, and elicit an undesired immune response. In contrast, adenoassociated viruses do not express viral proteins and are nonimmunogenic. They can infect dividing and nondividing cells without integrating into the genome and provide transient gene expression. Yet, adenoassociated viruses are difficult to construct because their development requires helper viruses that make purification problematic.
Retroviruses are the most extensively used vectors for applications in gene therapy. They are RNA viruses that, thanks to the viral enzyme reverse transcriptase, make a DNA copy of their genome, which can randomly integrate into the host cell genome. Replication-incompetent murine leukemia virus, which is nonpathogenic in humans, is the most used among retroviruses for gene therapy. Murine leukemia virus exists in host cells only as DNA and is unable to transcribe genes encoding viral coat proteins. Murine leukemia virus cannot elicit an immune response. As observed in recent clinical trials, however, the integrated retrovirus can disrupt the host DNA by insertional mutagenesis, producing serious adverse effects. In addition, because the virus is permanently integrated into the genome, the induced gene expression also is permanent. This phenomenon is not ideal for such transitory processes as periodontal regeneration and tissue regeneration in general.
Lentiviruses are a specialized form of retroviruses that have the advantage of infecting nondividing cells. Also, host genome integration seems to be more limited and less random than retroviruses, limiting the possibility of insertional mutagenesis. Lentiviruses are derived from pathogenic viruses, however, such as HIV-1, and may not be considered safe for clinical use. More importantly, they may provide a continuous expression of the transgene and may not be suitable for temporary regenerative processes.
For all the previously mentioned reasons, viral gene therapy may not be the most suitable gene therapy approach for tissue regeneration. Indeed, naked DNA for nonviral gene therapy is easier and cheaper to manufacture, is available on a large scale, is less immunogenic, presents a low likelihood of being inserted into the cell genome, and allows for transient expression of the transgene. Unfortunately, the transfection efficiency of naked DNA is extremely low and naked DNA remains elusive and ineligible for tissue regeneration and gene therapy in general. For this reason, several researchers have tried to complex the naked DNA in different chemical formulations to increase cellular DNA uptake and subsequent gene expression. In particular, cationic and anionic liposomes have been shown to increase the uptake of DNA in tissue culture cells and in vivo. Yet, as currently formulated, the transfection of cells by naked DNA is an inefficient process, with an estimated efficiency of 10 −9 that of viral vectors. Novel approaches have introduced the concept of passive uptake of naked DNA. By this method, the DNA molecules are immobilized in vivo by carriers, such as collagen or polymer matrices, to form the so-called “gene-activated matrices.” The immobilized DNA is then up-taken by the cells. Because the DNA stays in place for a longer time, the transfection efficiency is increased. By this technique, between 30% and 50% of the cell population exposed to the DNA expresses the transgene and the transfection is higher when compared with previous in vivo approaches.
Gene Therapy for Periodontal Regeneration
The efficacy of gene-based therapeutics for periodontal regeneration has been demonstrated only in preclinical studies, where viral approaches have been shown to have potential. Some of the most interesting studies in this field have been performed using viral vectors encoding for platelet-derived growth factor (PDGF). Initial investigations found that adenoviral vectors effectively transduced osteoblasts, cementoblasts, periodontal ligament cells, and gingival fibroblasts to induce the expression of PDGF in vitro. It was demonstrated that on viral transduction, the expression of PDGF was maintained for up to 10 days in human gingival fibroblasts. Subsequently, the feasibility of in vivo delivery of PDGF by adenoviral gene transfer to stimulate periodontal tissue regeneration in large tooth-associated alveolar bone defects in rats was demonstrated. An adenoviral vector encoding bone morphogenetic protein (BMP)-7 for regeneration of periodontal defects in rats was also successfully used, indicating that other growth factors may be effective in periodontal regenerative gene therapy.
Others have investigated the ability of adenoviral vectors to induce bone formation in bone defects. For instance, Lieberman and colleagues used an ex vivo approach to create BMP-2 producing bone marrow cells. The bone marrow cells producing BMP-2 were successfully used to heal a critical size femoral segmental defect in rats. Delivery of BMP-2 by adenoviral vector also proved to be successful in bone healing of segmental defects created in the femora of New Zealand rabbits and in regeneration of other bone defects. Recently, a combinatorial approach was successfully tested in cells transduced with adenoviruses encoding both BMP-2 and BMP-7. These cells were more effective in healing cranial defects than were cells individually transduced with adenoviruses encoding either BMP-2 or BMP-7.
Retroviruses have also been investigated as potential and effective vectors for bone regeneration. For instance, the ability of a retroviral expression vector encoding for sonic hedgehog to induce regeneration of bone was demonstrated in rat calvaria critical size defects. Retroviral vectors were also successfully used to transduce skeletal muscle cells, inducing them to express human BMP-2. These cells, once implanted into critical size calvaria defects in mice, induced a full closure of the defect by 8 weeks.
One of the most promising gene therapy approaches for periodontal regeneration is based on the combination of naked DNA with a biodegradable carrier. Using this approach, collagen or other carriers are used to engineer a so-called “gene-activated matrix” (GAM) that can carry and deliver the naked DNA in vivo. An elegant demonstration that this method can be used for bone regenerative therapy comes from the pioneering studies of Bonadio and coworkers. In their work, a plasmid encoding for the N-terminal fragment of the human parathyroid hormone was physically entrapped in a collagen matrix sponge and implanted into a critical size canine skeletal defect. This gene-activated collagen matrix induced bone regeneration in a stable, reproducible, and dose-dependent manner.
The GAM approach was later used by others for alternative gene therapy studies. For instance, plasmids that encode basic fibroblast growth factor-2, brain-derived neurotrophic factor, and neurotrophin-3 entrapped in GAM were shown to promote sustained survival of retinal ganglion cells for over 3 months after a central nervous system injury in rats. More recently, a study showed that angiogenesis and osteogenesis can be promoted by a GAM carrying a plasmid encoding for human vascular endothelial growth factor (VEGF) in atrophic bone nonunion defects in rabbits. Others have used a plasmid encoding transforming growth factors-ss1 entrapped in GAM fabricated with chitosan and gelatin to induce cartilage regeneration into cartilage defects of rabbit knee joints. In this study the authors achieved new cartilage formation and concluded that this therapeutic protocol provided a cost-effective, simple, and efficient method for repair of cartilage defects. Another recent work reports that modifying GAM with calcium-phosphate precipitates enhances the efficiency of the in vivo gene transfer. This study showed that the modified GAM carrying a plasmid encoding human BMP-2 is more efficient than the traditional GAM in inducing bone regeneration in a critical size segmental bone defect in rat tibiae.
Clearly, the nonviral approach using GAM has the potential to offer transient but efficient delivery of a specific gene that is suitable for periodontal regenerative therapy. Unfortunately, despite the high transfection efficiency observable, GAM still requires an enormous amount of plasmid DNA to achieve some therapeutic effect. The previously mentioned studies use plasmid amounts in the range of 1 to 100 mg, depending on the size of the bone defects. Moreover, difficulties in the preparation of collagen-based GAMs may limit its clinical use.
Gene therapy presents certain advantages when compared with other therapies. Because both cell transplantation and laboratory cell culturing are not needed, gene therapy may be safer and more cost-effective than cell-based therapies. Moreover, when compared with the existing recombinant single-protein–based therapies, gene therapy may mimic the complex natural process of periodontal tissue formation, because multiple genes, and multiple factors, can be delivered within the bone defect.
Since the inception of gene therapy (mid- to late-1980s), researchers have launched more than 400 clinical trials, testing the approach against a wide spectrum of diseases. The Food and Drug Administration, however, has recently declared that “little has worked” and more studies are needed to improve gene therapy. Despite some successes, several tragic outcomes, such as treatment-induced cancers and death caused by allergic reactions, have hampered and derailed the field. The encouraging findings of the previously mentioned studies may promote more much needed basic research with the final goal of developing effective and safer gene-based therapeutics.
Stem cells
Stem Cell Biology
The most relevant limitation of biomaterials available for tissue regeneration is their lack of promotion or delivery of competent cells to the site where tissue repair is required, resulting in inadequate regeneration. To overcome this problem, researchers are focusing their efforts on the use of stem cells to be delivered within the tissue defect.
A stem cell is a clonogenic (able to form colonies in cultures), undifferentiated cell that is capable of differentiating into a different phenotype. Stem cells that differentiate into any cell type from all the three germ layers of the body (ectoderm, endoderm, and mesoderm) are defined as “pluripotent” stem cells. In contrast, stem cells that can differentiate into a cell type from only one or two germ layers are defined as “multipotent.” In general, three categories of stem cells can be identified: (1) embryonic stem cells (pluripotent); (2) adult stem cells (multipotent); and (3) reprogrammed stem cells (either pluripotent or multipotent).
Embryonic stem cells are derived from the inner cell mass of the blastocyst. In culture they are able to form teratomas, agglomerations of cells with phenotypic characteristics of cell types from all the three germ layers of the body. There is still a plethora of concerns associated with their use in clinical therapies, including ethical concerns and biologic obstacles, such as their potential for tumorigenesis.
Adult stem cells have been isolated from many specialized tissues, including bone marrow, epithelium, adipose tissue, liver, and the nervous system, and may represent a safer approach to stem cell–based tissue regeneration. Because of their limited number and the technical difficulties related to their isolation and expansion, however, currently adult stem cells are used in few clinical applications. For instance, hematopoietic stem cells are used to treat lymphomas and bone marrow stromal stem cells have been shown to be successful in regenerating bone in animal and preclinical human studies.
Reprogrammed stem cells are defined as cells whose genetic program has been substituted or modified to induce a switch from one cell phenotype to another phenotype. Cell reprogramming is a challenging and yet fascinating goal for today’s regenerative medicine because reprogrammed cells may serve as a valuable and safe tool for tissue engineering.
There are four routes by which cell reprogramming can be achieved. The first, defined as nuclear transfer from somatic cells to eggs and oocytes, is achieved by transferring a viable cell nucleus into an enucleated egg ( Fig. 1 ). This method, first used for the creation of a cloned adult sheep (Dolly), remains ethically controversial for human studies because it requires the explantation and the nuclear transplantation of an unfertilized oocyte.
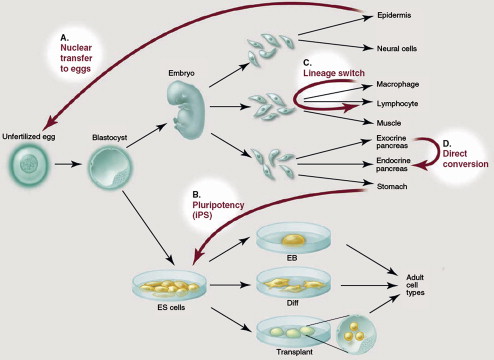
The second and more viable route is represented by the pluripotency induced in somatic cells by the overexpression of certain genes or by modulation of certain signaling pathways (see Fig. 1 ). Takahashi and Yamanaka at Kyoto University in Japan were the first to induce pluripotency in mouse fibroblasts by viral transduction of four genes named Oct 3/4 , Sox2 , c-Myc , and KLF4 . These cells, named “induced pluripotent” stem (iPS) cells, show properties similar to those of embryonic stem cells. Recent research has shown that Oct 3/4 alone may be sufficient to induce pluripotency in somatic cells. Opening the way to a safer approach to iPS cells, latest developments have shown the ability of nonviral methods, such as episomal vectors or plasmid vectors, to induce pluripotency in somatic cells. The modulation of certain signaling pathways induced by chemicals, such as valproic acid or other small molecules, is also being tested and may represent a safer alternative for the induction of iPS.
The third route to cell reprogramming is represented by lineage switching and is possible because of the discovery of so-called “master genes” (see Fig. 1 ). For instance, the overexpression of the gene MyoD can induce a nonmuscle cell back to a partially undifferentiated state and then guide its maturation into a muscle cell.
The fourth route is represented by direct conversion, a nonlineage switching of a cell into a different phenotype induced by specific genes (see Fig. 1 ). For instance, a recent study demonstrated the ability of three β-pancreas–specific genes to convert exocrine pancreas cells into insulin-producing pancreas β-cells.
The improvement of the iPS technology, of lineage switching, and of direct conversion technologies could represent the most exciting line of research for the development of effective, safe, and ethically acceptable stem cell–based therapies.
Stem Cells for Periodontal Regeneration
Cell-based therapeutics for periodontal tissue regeneration may be engineered thanks to the latest developments in stem cell research. Although science is far from the use of iPS cells for the regeneration of periodontal tissue, for clinical practice, the use of adult stem cells and of reprogrammed stem cells by lineage switching or direct conversion may represent a promising and valid avenue for the future of periodontal therapeutics. To this end, researchers have recently focused their attention on the isolation and characterization of periodontal stem cells as common progenitors of fibroblasts, cementoblasts, and osteoblasts that form the tissues of the periodontium. For several years it has been known that cells derived from the periodontal ligament can differentiate into osteoblasts or cementoblasts to contribute to periodontal regeneration. Direct evidence of the existence of a stem cell population within the periodontal tissue was found in 2004, however, as multipotent periodontal ligament stem cells (PDLSC) were first isolated from the periodontal ligament of extracted teeth. These cells express mesenchymal stem cell markers, such as STRO-1 and MCAM (CD146), and were shown to be clonogenic and able to differentiate into adipocytes, osteoblasts, and cementoblast-like cells both in vitro and in vivo. Using antibodies against STRO-1, MCAM, and CD44 Chen and colleagues have identified and localized PDLSC in diseased and healthy periodontal human tissues. Recently PDLSC were also indentified in regenerating periodontal tissue, indicating that they directly contribute to periodontal regeneration. PDLSC are very similar to other adult stem cells in that they are multipotent and clonogenic and express common markers, such as STRO-1 and MCAM. This similarity may also be detrimental, however, when it comes to isolation and expansion of PDLSC for the construction of cell-based therapeutics. This is because the use of antibodies against STRO-1 and MACM may result in the selection of a heterogeneous population of mesenchymal stem cells rather than a pure population of PDLSC. The challenge lies in the ability of identifying a PDLSC-specific marker that allows for the selection of a pure PDLSC population. Toward this end, researchers have been testing if these cells express markers, such as scleraxis, a tendon-specific transcription factor that may be expressed in higher concentrations in PDLSC because of their ability to form a ligament. Although PDLSC do express scleraxis in higher concentration when compared with bone-marrow derived and dental-pulp derived stem cells, it is clear that this is not a unique marker for PDLSC.
Other efforts are under way to find an appropriate delivery system for engineering an efficient cell-based therapeutic tool for periodontal tissue regeneration. Flores and colleagues have found that fibrin gels carrying several layers of PDL cells (the so-called “cell sheet technique”) can be successfully used as a delivery system for these cells, whereas Nakahara and colleagues have successfully tested collagen sponge scaffolds seeded with PDL cells for the regeneration of periodontal fenestration defects in beagle dogs. Additionally, a delivery system for PDLSC based on a combination of bovine bone with human dentin was shown to be effective because these stem cells formed a cementum-like complex in subcutaneous dorsum pockets of immunocompromized mice. Recently, platelet-rich plasma was also proposed as a putative carrier for adult stem cells into periodontal defects and into larger bone defects.
Enormous steps forward have been taken in the research of periodontal stem cells and cell delivery systems, leading to the promise of cell-based therapeutics for periodontal regeneration in the near future.
Stem cells
Stem Cell Biology
The most relevant limitation of biomaterials available for tissue regeneration is their lack of promotion or delivery of competent cells to the site where tissue repair is required, resulting in inadequate regeneration. To overcome this problem, researchers are focusing their efforts on the use of stem cells to be delivered within the tissue defect.
A stem cell is a clonogenic (able to form colonies in cultures), undifferentiated cell that is capable of differentiating into a different phenotype. Stem cells that differentiate into any cell type from all the three germ layers of the body (ectoderm, endoderm, and mesoderm) are defined as “pluripotent” stem cells. In contrast, stem cells that can differentiate into a cell type from only one or two germ layers are defined as “multipotent.” In general, three categories of stem cells can be identified: (1) embryonic stem cells (pluripotent); (2) adult stem cells (multipotent); and (3) reprogrammed stem cells (either pluripotent or multipotent).
Embryonic stem cells are derived from the inner cell mass of the blastocyst. In culture they are able to form teratomas, agglomerations of cells with phenotypic characteristics of cell types from all the three germ layers of the body. There is still a plethora of concerns associated with their use in clinical therapies, including ethical concerns and biologic obstacles, such as their potential for tumorigenesis.
Adult stem cells have been isolated from many specialized tissues, including bone marrow, epithelium, adipose tissue, liver, and the nervous system, and may represent a safer approach to stem cell–based tissue regeneration. Because of their limited number and the technical difficulties related to their isolation and expansion, however, currently adult stem cells are used in few clinical applications. For instance, hematopoietic stem cells are used to treat lymphomas and bone marrow stromal stem cells have been shown to be successful in regenerating bone in animal and preclinical human studies.
Reprogrammed stem cells are defined as cells whose genetic program has been substituted or modified to induce a switch from one cell phenotype to another phenotype. Cell reprogramming is a challenging and yet fascinating goal for today’s regenerative medicine because reprogrammed cells may serve as a valuable and safe tool for tissue engineering.
There are four routes by which cell reprogramming can be achieved. The first, defined as nuclear transfer from somatic cells to eggs and oocytes, is achieved by transferring a viable cell nucleus into an enucleated egg ( Fig. 1 ). This method, first used for the creation of a cloned adult sheep (Dolly), remains ethically controversial for human studies because it requires the explantation and the nuclear transplantation of an unfertilized oocyte.
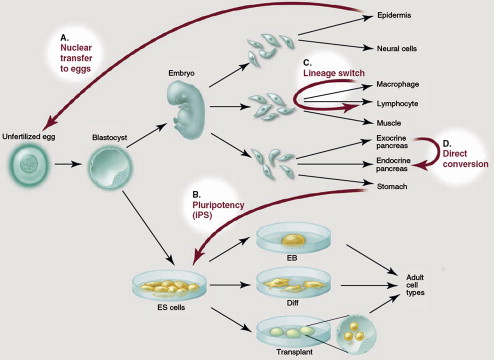
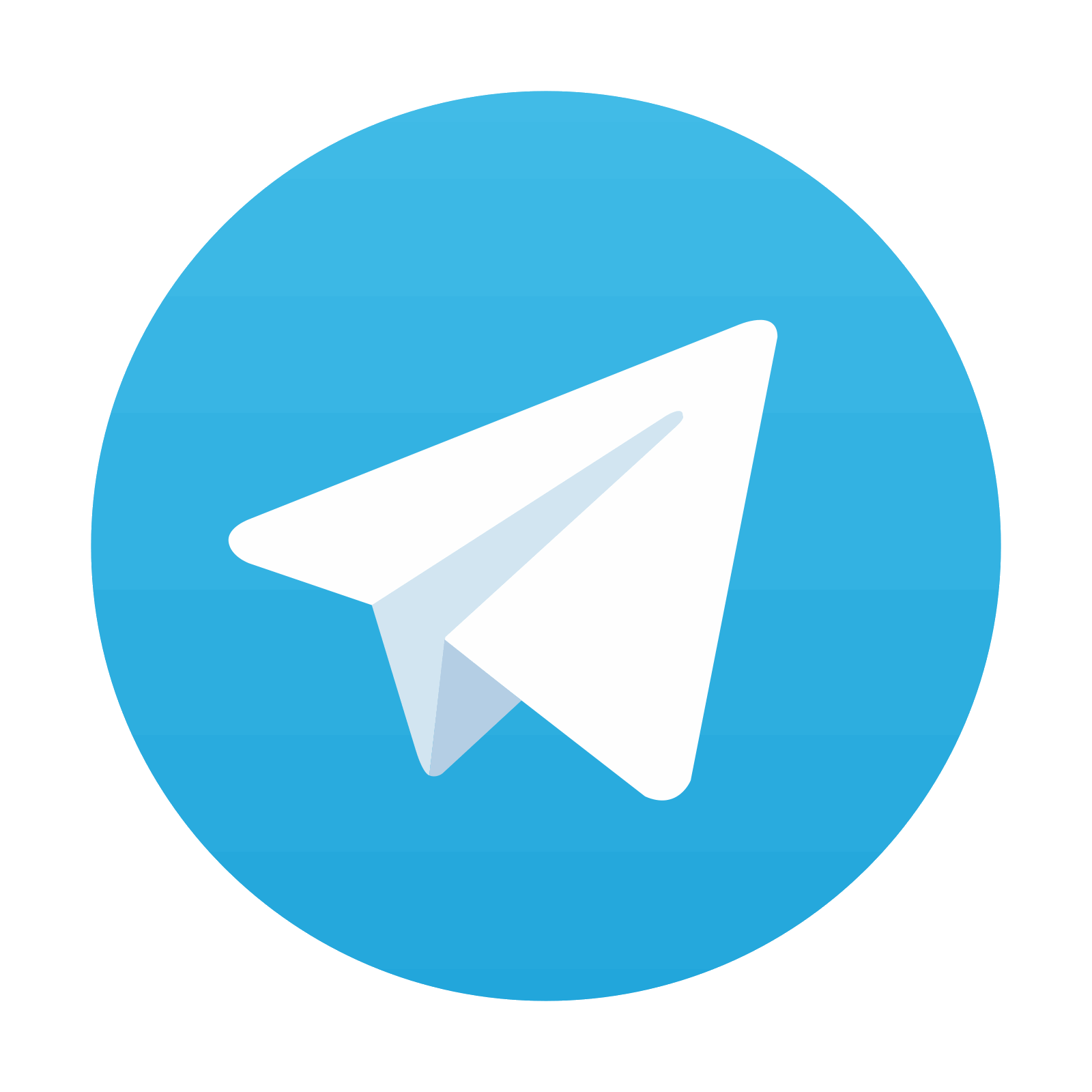
Stay updated, free dental videos. Join our Telegram channel

VIDEdental - Online dental courses
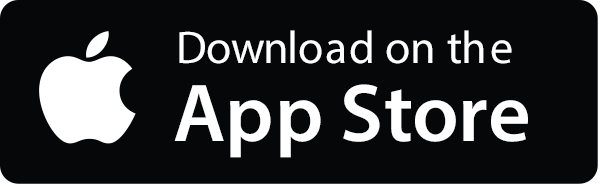
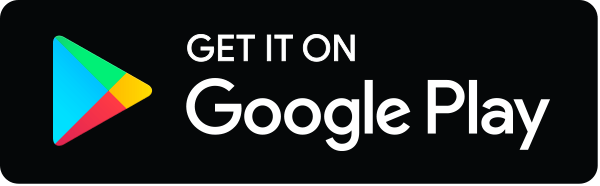