Fig. 15.1
Dental fluorosis. (a) Mild fluorosis with slight accentuation of the perikymata, (b) moderate fluorosis, showing a white opaque appearance, (c) moderate, white opaque enamel with some discoloration and pitting, (d) severe fluorosis [with permission from a reference (Denbesten and Li, 2011), Fig. 1] DENBESTEN, P. & LI, W. 2011. Chronic fluoride toxicity: dental fluorosis. Monogr Oral Sci, 22, 81–96
15.1.1 Mechanisms of Enamel Fluorosis
Studies to determine the mechanisms by which fluoride results in dental fluorosis have used animal models. The most frequently used model to study fluorosis mechanisms is the rodent incisor, as it is not possible to do similar studies using human teeth. Fluoride can be given in drinking water beginning at 21 days, when rodents are weaned. At this time, the incisors are fully erupted; however, because the rodent incisor is a continuously erupting tooth, all stages of enamel formation are present, so that the effect of fluoride at each stage of enamel formation can be investigated.
Models to Study the Fluorosis Mechanisms Should Be Based on Comparable Concentrations to Those Found in Human Serum
A difference between rodents and humans, which sometimes results in confusion as to the relevance of studies of fluoride mechanisms, is the fact that rodents must consume approximately 10 times the amount of fluoride in drinking water than humans to result in the same serum fluoride level and degree of fluorosis. For example, humans drinking 3–5 ppm fluoride in water (1 ppm F = 52.6 μM) have serum fluoride levels of 3–5 μM (Guy et al. 1976) which form fluorosed enamel. For a mouse to have similar serum fluoride levels, the mouse must ingest drinking water containing approximately 50 ppm F (Zhang et al. 2014). It is not known why rodents require 10 times the concentration of fluoride in their drinking water to have serum fluoride levels similar to humans. However, the fact remains that comparable serum fluoride levels should be used to assess the biological relevance of an animal model. Much confusion has resulted in interpretation of studies on fluorosis mechanisms because of these concentration differences in fluoride ingested in water by rodents as compared to humans. Because of this, the relevance of rodent studies to fluorosis mechanisms in humans has been questioned and their relevance not clearly understood.
Similar issues relating to the relevance of the fluoride concentration used occur in vitro of fluoride mechanisms. Many in vitro studies expose cells to fluoride levels found in drinking water, which are in fact 50 times higher than would be found in serum. For example, 1 ppm F, which is equivalent to 52.6 μM F, ingested by humans in drinking water, results in serum fluoride levels of approximately 1 μM serum F. This suggests that the use of 1 ppm fluoride cell culture in vitro would actually represent fluoride levels approximately 50 times that likely are to be found at the cellular level in vivo, and the results obtained are not likely to be relevant to in vivo fluoride exposure to humans. Therefore, to understand the mechanisms by which fluoride may affect both enamel formation and other cells and tissues, experiments conducted and the interpretation of their results must be done with an understanding of biological relevance.
Fluoride Exposure Causes Both Hyper- and Hypomineralization of the Enamel Matrix
Fluoride is a highly electronegative anion and, as such, enhances mineral formation. This local hypermineralization in the enamel matrix depletes the local reservoir or free calcium ions and then may also act as a physical barrier, impairing diffusion of ions and peptides, to result in a subsequent band of hypomineralized enamel (Guo et al. 2015). Therefore, within the matrix, it is possible that a more rapid mineral precipitation in the presence of fluoride may result in the presence of hypermineralized bands followed by hypomineralized bands in the enamel matrix (Bronckers et al. 2009).
Fluoride Exposure May Increase Acidification of the Developing Enamel Matrix
Formation of hydroxyapatite biominerals (HAP) results in the formation of protons (10Ca2+ + 6 HPO4 2− + 2H2O → Ca10 (PO4)6 (OH)2 + 8H+), which acidify the enamel matrix. Therefore, regulation of matrix pH by ameloblasts is crucial for sustained crystal growth. In the presence of fluoride, increased mineral formation may result in local acidification, which is buffered by amelogenin proteins in the enamel matrix at the secretory stage (Guo et al. 2015).
At the maturation stage, where the most of the enamel HAP mineralization occurs, the pH in the enamel matrix changes periodically between acidic (pH 5.8) and neutral (pH 7.2) (Sasaki et al. 1991; Smith 1998). Protons generated during HAP formation in the maturation stage are neutralized by bicarbonate secreted from the apical end of ruffled-ended maturation ameloblasts through anion exchangers of the Slc26a family (Jalali et al. 2014). Transmembrane proteins, including cystic fibrosis transmembrane conductance regulator, anion exchanger-2, carbonic anhydrase-2, and sodium hydrogen exchanger-1, are involved in this pH regulation mechanism (Guo et al. 2015; Lyaruu et al. 2008; Alper 2009; Concepcion et al. 2013). In the fluorosed enamel matrix, retention of amelogenin protein may also buffer pH changes in the matrix, secondary to mineral formation, thereby reducing the acidification of the matrix under ruffle-ended ameloblasts (Guo et al. 2015).
Bicarbonate is exchanged for chloride (Cl-) in the matrix, and with increased secretion of bicarbonate, the amount of chloride in the mineralizing enamel matrix is decreased. The reduced amount of Cl found in the fluorosed enamel matrix supports the hypothesis that increased enamel matrix acidification and subsequent neutralization occurs in the presence of increasing amounts of fluoride (Bronckers et al. 2015). Recent evidence has shown that maturation stage ameloblast modulation depends on Cl- (Bronckers et al. 2015), and therefore reduced Cl in the enamel matrix may be responsible for the fewer number of ameloblast modulations from ruffle-ended to smooth-ended ameloblasts, found in the presence of fluoride.
Amelogenins Are Retained in Fluorosed Maturation Stage Enamel
Fluorosed maturation stage enamel is characterized by a delay in the removal of amelogenin matrix proteins (DenBesten and Crenshaw 1984; Wright et al. 1996). It is possible that this delay in removal of amelogenins is due to altered ameloblast modulation (DenBesten et al. 1985; Smith et al. 1993). Other factors that may contribute to the retention of amelogenins in fluorosed enamel include increased binding of amelogenins to the fluoride-containing HAP crystals (Tanimoto et al. 2008), which may delay the removal of amelogenins from the enamel matrix. Recent studies in mice have also shown a reduced expression of KLK4, the proteinase responsible for hydrolyzing amelogenins and other matrix proteins in the maturation stage (Suzuki et al. 2014). A reduction in KLK4 could also delay the hydrolysis of amelogenins and their removal from the enamel matrix, resulting in less final mineral formation and a more hypomineralized enamel matrix. These effects of fluoride at the maturation are consistent with the observation that fluoride-induced subsurface hypomineralization can independently occur in the maturation stage, even without prior exposure to secretory stage enamel. However, fluorosis is most severe with fluoride exposure to all stages of enamel formation (DenBesten et al. 1985; Richards et al. 1985; Suckling et al. 1988).
Fluoride Can Alter Cell Function
Amelogenesis is a dynamic interaction between the differentiating ameloblasts and the self-assembling mineralized matrix. The primary mechanisms responsible for the formation of fluorosed enamel appear to be related to effects on matrix mineralization. However, it is possible that fluoride may, to a lesser extent, directly affect cell function, though it is difficult to differentiate direct cellular effects from those caused by changes in the mineralizing matrix. Some studies have found that fluoride appears to alter the timing of gene expression in ameloblasts overlying fluorosed as compared to control enamel, possibly related to a fluoride-related enhancement of Gαq activity in secretory ameloblasts (Zhang et al. 2014).
15.1.2 Fluoride Effects on Dentin Formation
The dentin matrix is far less mineralized than the enamel matrix and is comparatively unaffected by fluoride. However, in vitro studies of mildly and moderately fluorosed human dentin, showing increased caries susceptibility (Waidyasekera et al. 2007), suggest that fluoride also alters dentin biomineralization. In support of this possibility, reduced dentin microhardness and increased dentin fluoride were shown to correlate enamel fluorosis severity (Vieira et al. 2005). Fluorosed dentin has been characterized as having increasingly disorganized dentin crystals (Kierdorf et al. 1993; Vieira et al. 2004, 2005; Nelson et al. 1989; Yaeger 1966; Waidyasekera et al. 2010), and structurally, severely fluorosed human dentin is described as the distinct layering of hypomineralized lines and extensive areas of interglobular dentin (Fejerskov et al. 1977), with the irregular and densely arranged dentinal tubules (Rojas-Sanchez et al. 2007). These studies indicate that fluoride-related changes can occur while primary dentin is formed.
15.2 Fluoride in Food and Water
Water Fluoridation
One of the first attempts to provide effective caries control on a population basis was through the use of artificial water fluoridation programs. By the 1940s, there was a substantial body of literature comparing the prevalence and severity of dental caries among populations living in communities with differing levels of fluoride in the water. These studies showed that dental caries levels dropped sharply as water fluoride levels rose to 1.0 ppm. This was also the concentration at which prevalence of dental fluorosis began to increase in these same populations (Dean 1938). As a result of this work, 1 ppm F was determined to be the point at which one could expect to receive an optimal benefit with minimal side effects of dental fluorosis.
On January 25, 1945, Grand Rapids, Michigan, USA, became the first city in the world to adjust its water fluoride concentration to a level expected to reduce dental caries, and 10 years later, the study found that though mild forms of dental fluorosis were increased, dental caries was significantly decreased (Arnold et al. 1956). Other studies have continued to confirm the benefits of water fluoridation, though more recently the overall rates of caries were found to be dropping in the population at large (Brunelle and Carlos 1990). Caries rates throughout the world have followed similar trends, and interestingly, caries rates have decreased whether or not the local water supplies are fluoridated, most likely related primarily to the widespread worldwide expansion of the use of fluoride toothpaste after 1970. Recently, a Cochrane systematic review that evaluated the effectiveness of water fluoridation for the prevention of caries suggested the need to evaluate all sources of fluoride before such systems should be considered. The review concluded, “The decision to implement a water fluoridation programme relies upon an understanding of the population’s oral health behavior (e.g., use of fluoride toothpaste), the availability and uptake of other caries prevention strategies, their diet and consumption of tap water and the movement/migration of the population.” However, there was a significant association between dental fluorosis and level of exposure to fluoride (Iheozor-Ejiofor et al. 2015). In spite of the issues raised regarding fluoride, the use of fluoridated water has provided significant anticaries benefits. The American Dental Association, the US Center for Disease Control, and the World Health Organization all support water fluoridation. According to the (http://www.who.int/water_sanitation_health/oralhealth/en/index2.html), “Fluoridation of water supplies, where possible, is the most effective public health measure for the prevention of dental decay.”
Salt Fluoridation
A key objection to community water fluoridation is that it fails to provide consumers with a choice, as the only alternative is for consumers to purchase bottled water that is not fluoridated. One alternative to water fluoridation is salt fluoridation. To some, the use of salt fluoridation over water fluoridation is an attractive option. Fluoridated salt is generally sold side by side on grocery shelves with non-fluoridated salt; the choice is up to the individual consumer as to which one they prefer.
Originally introduced in 1955 in Switzerland as an extension of programs that utilized iodized salt for the prevention of thyroid conditions (Burgi and Zimmermann 2005), the fluoridation of table salt has been demonstrated to provide caries reductions on par with water fluoridation programs (Marthaler 2005) when the majority of the salt consumed is fluoridated. Fluoride concentrations in salt ranging from 90 mg/kg up to 350 mg/kg have been tested, with some studies suggesting the level of 250–300 mg/kg as being optimal. One study demonstrated that salivary fluoride levels after eating a meal prepared with salt fluoridated at 250 mg/kg were similar to those of individuals exposed to water fluoride levels of 1 mg/l (Hedman et al. 2006). A level of 200 mg/kg is considered the minimum level necessary to provide a reasonable caries benefit (Sampaio and Levy 2011), suggesting that salt fluoridation can result in increased salivary fluoride.
Human studies that assessed the effectiveness of salt fluoridation in Columbia, Hungary, and Switzerland confirmed the effectiveness of this approach, demonstrating results that were not unlike those seen with early water fluoridation programs (Marthaler and Petersen 2005; Jones et al. 2005). Fluoridated salt is relatively easy to deliver to consumers through a range of channels, including the use of domestic salt, participation in school meal programs, and bread made in local bakeries. One of the advantages to the use of salt fluoridation is its availability in areas where fluoridated toothpastes are not broadly available or are considered to be too expensive. However, when combined with the use of fluoridated toothpastes, fluoride exposures may reach above optimal levels (Baez et al. 2010).
One example that highlights the effectiveness of fluoridated salt comes from Jamaica, where virtually all salt intended for human consumption has been fluoridated since 1987 (Jones et al. 2005). Although fluoride toothpastes have been available there since 1972, an oral health survey conducted in 1984 showed extremely high caries rates in Jamaican children, with the assumption being that toothpastes were generally not used on a regular basis (Table 15.1). In 1986, the Jamaica Parliament approved a salt fluoridation program, as water fluoridation was deemed to be technically unfeasible in the region. The natural concentration of fluoride in the water was less than 0.3 mg/ml. At the time, Jamaica had only one supplier of salt, which made salt fluoridation a viable option. Salt was fluoridated at 250 mg/kg, using potassium fluoride, with technical guidance provided by the Pan American Health Organization (PAHO). Urinary excretion studies, which are commonly used to monitor excessive ingestion of fluoride (Marthaler and Schulte 2005) conducted at baseline and after 20 months of Jamaica’s salt fluoridation program indicated fluoride concentrations were no greater than those that would be expected for a temperate climate where water fluoridation programs were in place. A follow-up survey in 1995 confirmed the effectiveness of the program, with dramatic reductions in caries noted in each of the age groups measured (Table 15.1).
Table 15.1
Mean number of decayed, missing, or filled permanent teeth (DMFT) in Jamaican children, 1984 and 1995
Age (years)
|
Mean number of DMFT
|
Percent decrease in DMFT
|
|
---|---|---|---|
1984
|
1995
|
||
6
|
1.71
|
0.22
|
87 %
|
12
|
6.72
|
1.08
|
84 %
|
15
|
9.60
|
3.02
|
68 %
|
Salt fluoridation is broadly available in many Latin American countries, with the exception of Brazil, Chile, and Panama, where fluoride toothpastes are commonly used. One issue regarding the exclusive use of fluoridated salt is the potential for erratic exposure; usage can vary significantly from one individual to another. Another issue is the lack of standardized processes for fluoridated salt in countries where there are multiple producers of salt, with no effective surveillance mechanisms in place. From an economic viewpoint, salt fluoridation appears to be a cost-effective measure, with one report indicating the per capita costs average between 0.015 and 0.030 (USD) per year (Gillespie and Marthaler 2005). However, recent reviews point to the lack of available, randomized clinical trials comparing salt fluoridation to other methods of caries prevention (Espelid 2009; Cagetti et al. 2013).
Milk Fluoridation
In addition to salt and water fluoridation programs, milk fluoridation is another approach that is used in some geographic areas. Like water fluoridation, this approach does not require a change in consumer behaviors in order to provide an anticaries benefit. The basic premise is that ingestion of fluoridated milk will maintain salivary fluoride levels at levels similar to those achieved in individuals living in areas of optimally fluoridated water systems.
While individual trials have suggested significant benefits associated with milk fluoridation programs (Rusoff et al. 1962; Stephen et al. 1984), systematic reviews of fluoridated milk have concluded that there is a lack of well-controlled randomized clinical trials to confirm the effectiveness of this approach (Espelid 2009; Cagetti et al. 2013; Yeung et al. 2005, 2015). Though some effectiveness has been shown for primary teeth (Cagetti et al. 2013), as noted in a recent Cochrane review: “There is low quality evidence to suggest fluoridated milk may be beneficial to schoolchildren, contributing to a substantial reduction in dental caries in primary teeth. Additional randomized clinical trials of high quality are needed before we can draw definitive conclusions about the benefits of milk fluoridation”(Yeung et al. 2015).
15.3 Fluoride and Remineralizing Agents
Fluoride in the Biofilm
Dental caries occurs when bacteria in a biofilm produce lactic acid by saccharolytic fermentation. This acid can penetrate through to the tooth surface that is protected by pellicle, a natural protective protein barrier, and dissolve the hydroxyapatite crystals in subsurface enamel, resulting in the formation of subsurface lesions (Levine 2011; Amaechi and van Loveren 2013; Buzalaf et al. 2011). If fluoride is present in the plaque fluid when bacteria produce acids, it will penetrate along with the acids through the plaque subsurface and adsorb to apatite crystal surfaces. When the pH returns to pH 5.5 or above, the saliva, which is supersaturated with calcium and phosphate, provides calcium and phosphate to bind to the fluoride ions and form fluorapatite mineral, which is relatively less acid soluble than the carbonated hydroxyapatite mineral of a natural tooth (Amaechi and van Loveren 2013; Buzalaf et al. 2011; Stoodley et al. 2008).
Fluoride, which is a single, highly electronegative ion, operates via two primary mechanisms: inhibiting enamel demineralization and enhancing the natural process of enamel remineralization (ten Cate and Featherstone 1991). In addition, fluoride can be incorporated into bacterial biofilms and, if present at high enough concentrations, can inhibit enolase (Qin et al. 2006). Enolase catalyzes the production of phosphoenolpyruvate, a precursor of lactic acid from 2-phosphoglycerate, during glycolysis. Oral bacteria utilize the phosphoenolpyruvate transport system to transfer mono- and disaccharides into the cytosol. Fluoride not only inhibits lactic acid production but also the phosphoenolpyruvate transport system-mediated uptake of saccharide substrates.
Of importance to both of the primary mechanisms of action for fluoride is the transport of fluoride through the biofilm to the enamel surface. Studies of the transport of fluoride through the biofilm are conflicting. One group of researchers showed that exposure of enamel to NaF (1000 ppm F-) for 30 or 120 s (equivalent to toothbrushing) or for 30 min, resulted in increased plaque fluoride concentrations near the saliva interface, while concentrations near the enamel surface remained low. Fluoride penetration increased with duration of NaF exposure, and removal of exogenous fluoride resulted in fluoride loss and redistribution. The authors concluded that penetration of fluoride into plaque biofilms during brief topical exposure is restricted, which may limit anticaries efficacy (Watson et al. 2005). However, another study showed that following the use of a 0.2% fluoride rinse, fluoride penetrated through the biofilm, causing some effect on the viability of the biofilm mass (Rabe et al. 2015). Although there are questions that still need to be answered with respect to how fluoride impacts the biofilm, it is clear that both the application and the retention of fluoride in plaque, plaque fluids, and oral tissue reservoirs play important roles in overall effectiveness of fluoride (Zero 2006).
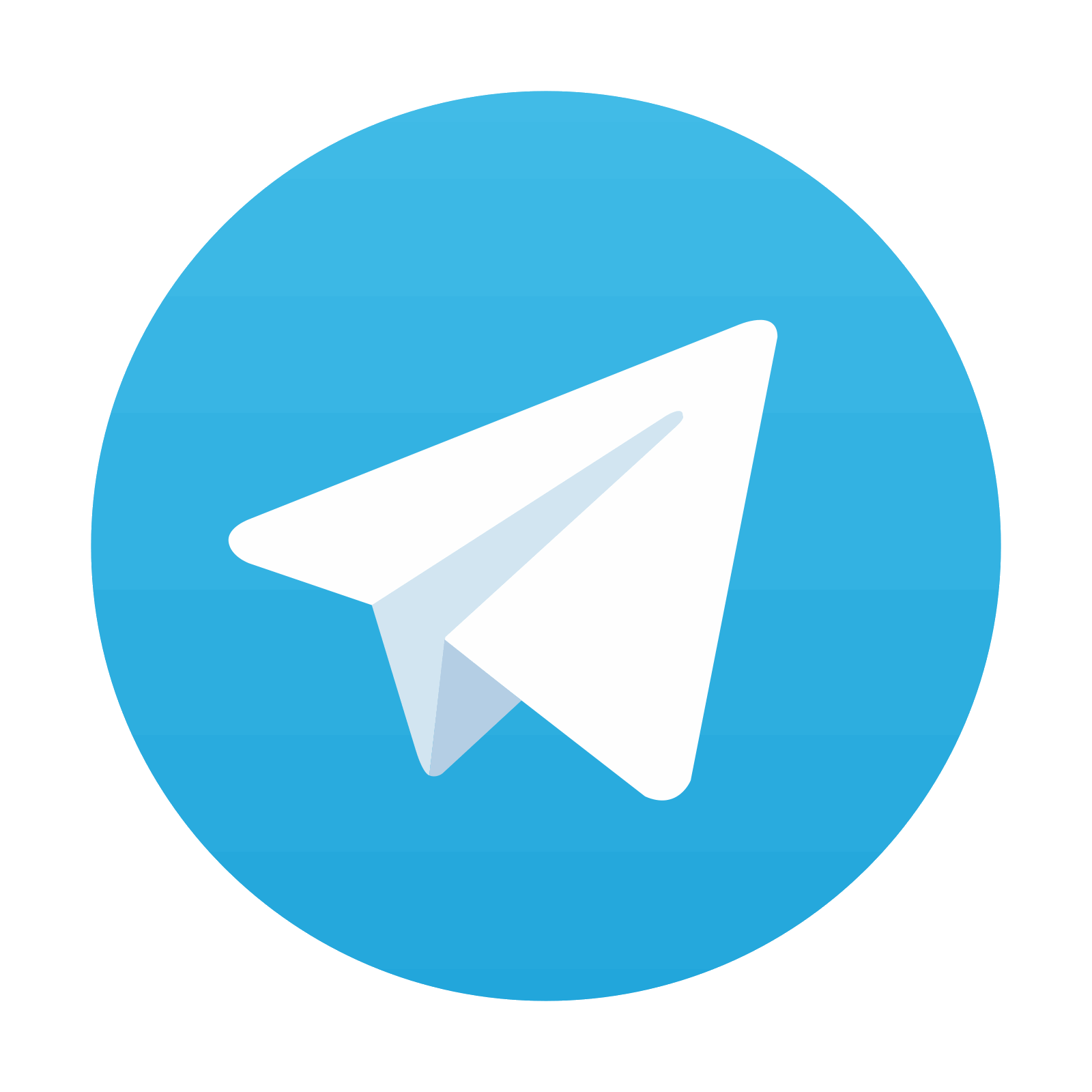
Stay updated, free dental videos. Join our Telegram channel

VIDEdental - Online dental courses
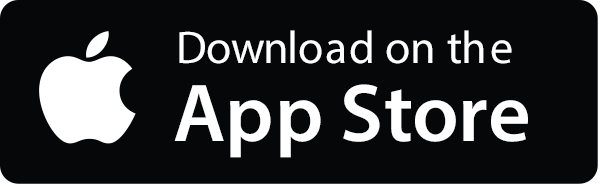
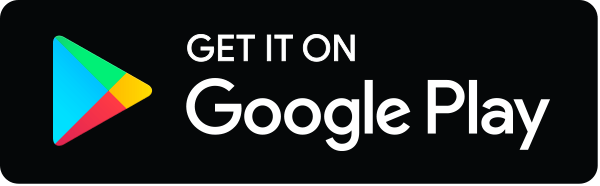