Abstract
Objectives
To evaluate the effects of silica (SiO 2 ) (0.5 wt%) and zinc oxide (ZnO) (0.25 wt%) dopants on the mechanical and biological properties of tricalcium phosphate (TCP) scaffolds with three dimensionally (3D) interconnected pores.
Methods
Scaffolds were created with a commercial 3D printer. Post sintering phase analysis was determined by X-ray diffraction. Surface morphology of the scaffolds was examined by field emission scanning electron microscopy (FESEM). Mechanical strength was evaluated with a screw driven universal testing machine. MTT assay was used for cellular proliferation characteristics and cellular morphology was examined by FESEM.
Results
Addition of dopants into TCP increased the average density of pure TCP from 90.8 ± 0.8% to 94.1 ± 1.6% and retarded the β to α phase transformation at high sintering temperatures, which resulted in up to 2.5 fold increase in compressive strength. In vitro cell–materials interaction studies, carried out using hFOB cells, confirmed that the addition of SiO 2 and ZnO to the scaffolds facilitated faster cell proliferation when compared to pure TCP scaffolds.
Significance
Addition of SiO 2 and ZnO dopants to the TCP scaffolds showed increased mechanical strength as well as increased cellular proliferation.
1
Introduction
Currently in dental applications, synthetic materials such as amalgam, resin, gutta-purcha, dentures and metal implants have been used to treat conditions such as caries, pulpits, periodontal disease and trauma. These repair methods are incapable of reproducing the natural function of the lost tissue and lead to mechanical deficiencies and reduced tooth vitality . Long term studies have shown that these treatments may have an effect on a patient’s masticatory and digestive functions . In the past 15 years, due in part to the shortcomings of these materials, there has been a growing interest in the investigation of synthetic bone alternatives as well as manufacturing methods. Advances in rapid prototyping give rise to the possibility of designing patient specific implants with complex geometry that can be tailored to the patient’s defect site . A scaffold may be used to grow complex tissues such as dentin and pulp when seeded with a suitable cell line .
Bone and dental substitution materials should encompass many different characteristics for consideration in clinical use. Not only should the material be porous to serve as a scaffold for capillary growth, the material should have excellent biocompatibility, osteoconductivity, and a complete lack of antigenicity . Ideally, a scaffold will offer mechanical support and will be resorbed as new bone and tissue growth occur. Calcium phosphate (CaP) materials have been widely studied for their use in orthopedic and dental implant applications and tissue scaffolds due to their excellent biocompatibility, controllable bioresorbability and compositional similarity to bone . Characteristics of scaffolds such as density, pore shape and size and pore interconnectivity are important parameters that will manipulate tissue ingrowth and mechanical interlocking at the implant–bone interface . Many methods for creating complex CaP scaffolds have been investigated including direct and indirect extrusion freeforming, selective laser sintering, stereolithography and ink-jet printing . Direct ink-jet printing has demonstrated very good resolution with great control over the complexity of geometry . Other advantages of 3D printing are that macroporosity is induced into the scaffold during the fabrication process without the need for a support structure . Moreover, these scaffolds are fabricated directly using the 3D printer with designed materials unlike many other processes where multiple post processing steps are needed.
One of the major disadvantages of ceramic scaffolds is their low strength, especially at high volume percentages of porosity . Recent studies have shown that by doping β-TCP with trace elements commonly found in bone, the dissolution/resorption rates, densification, cell–material interaction and mechanical strength can be controlled . One study showed that in osteoporotic subjects, supplemental silicon and monomethyl trisilanol resulted in increases in femoral and lumbar spine bone mass density . The same study demonstrated that this treatment was more effective than a bisphosphonate (Etidronate) and sodium fluoride treatment. Silicon has been proven to be an important trace element in bone and connective tissue formation and can stimulate biological activity by increasing the solubility of the material, generating a more electronegative surface and creating a finer microstructure resulting in transformation of the material surface to a biologically equivalent apatite . Zinc, also an important trace element in bone formation, has been shown to control grain growth, increase density and have stimulatory effects on bone formation when added to CaP . Zinc is released during skeletal breakdown and has shown to inhibit osteoclastic bone resorption . It does this by inhibiting osteoclast-like cell formation from bone marrow cells and inducing apoptosis of mature osteoclast . Osteoporotic patients have also been shown to have lower levels of skeletal zinc than that of control groups . This study examines the effects of silica (SiO 2 ) and zinc oxide (ZnO) addition, as a binary dopant system, on the physical, mechanical and biological properties of 3D interconnected porous TCP scaffolds created by the 3-D printing, a solid freeform fabrication method.
2
Materials and methods
2.1
Fabrication of porous scaffolds
High purity oxide based sintering additives, silicon dioxide (SiO 2 ) (99%+ purity) and zinc oxide (ZnO) (99.9%+ purity) were purchased from Fisher Scientific (Fair Lawn, NJ). Synthetic β-tricalcium phosphate powder was obtained from Berkley Advanced Biomaterials Inc. (Berkeley, CA) with an average particle size of 550 nm and specific average surface area of 10–50 m 2 g −1 . Powder was made in batches of 100 g and doped with 0.25 wt% ZnO and 0.5 wt% SiO 2 as a binary dopant system. Dopant amounts were chosen based on previous research from our group . Powder was added to and mixed in 500 mL polypropylene Nalgene bottles, with 300 g of 5 mm diameter zirconia milling media. 150 mL of ethanol was added and ball milling was carried out for 6 h at 70 rpm to minimize the formation of agglomerates and increase the homogeneity of the powder. After milling, the Nalgene bottle was placed in an oven at 60 °C for 24 h for drying. Milling media were removed and the powder was further dried at 60 °C for another 24 h. Finally, agglomerates were removed using a mortar and pestle.
Cylindrical scaffold CAD (Computer Aided Design) files (diameter 7 mm and height 10.5 mm) were created with interconnected square channels of 1000 μm, 750 μm, and 500 μm sides ( Fig. 1 (a) ). Scaffolds were fabricated using a 3-D printer (R-1 R&D printer by ProMetal). The printing system consists of a deposition bed that starts with only a small base layer of powder, a feed bed that is filled with powder, a powder spreader, an ink jet print head and a drying unit. A schematic representation of operation is given in Fig. 1 (b). The printer head sprays binder onto the loose powder in the deposition bed according to the CAD pattern, the deposition bed is lowered by 20 μm and the feeder bed is raised by 60 μm. The spreader will then push the excess powder from the feeder bed and evenly spread it onto the lowered deposition bed. The process is repeated, layer by layer, until the CAD image is completely printed. Parts were built in 20 μm layers thickness with commercial organic water based binder obtained from ProMetal (Irwin, PA). Once finished, parts were dried at 150 °C for 1.5 h. Green scaffolds were then gently brushed clean and the remaining powder was removed by using a compressed air blower. After cleaning, green scaffolds were sintered in a muffle furnace at 1250 °C for 2 h. Important fabrication parameters that were optimized include binder selection, binder drop size and amount deposited, powder particle size, drying time between layers, powder spread rate, build layer thickness and powder feed to build ratio.

2.2
Microstructure, phase analysis and mechanical properties
The surface morphologies of the sintered scaffolds were observed under a field emission scanning electron microscope (FESEM) (FEI Inc., OR, USA). Phase analysis of sintered β-TCP samples with and without dopants was carried out by X-ray diffraction (XRD) using a Siemens D-500 X-ray powder Diffractometer (Siemens AG, Karlsruhe, Germany) with CuK α radiation and a Ni-filter. Each run was performed with 2 values between 10° and 60° at a step size of 0.02° and a count time of 0.5 s per step. Density was measured using Archimedes method. Samples were weighed initially dry and then submerged in boiling water for 3 min to remove any excess air that may be trapped in the porous structure. The samples were then transferred from the boiling water to room temperature water, where the weight was recorded again ( n = 3). Compressive strengths of undoped and doped TCP scaffolds were determined using a screw-driven universal testing machine (AG-IS, Shimadzu, Japan) with a constant crosshead speed of 0.33 mm/min. Compressive strength was calculated using the maximum load recorded and the sample dimensions. Compressive strength was tested on ten samples for each composition.
2.3
In vitro osteoblast cell–material interactions
Pure and doped TCP samples were studied for their cell–materials interactions using established human fetal osteoblast (hFOB) cells (hFOB 1.19, ATCC, Manassas, VA). The base medium for this cell line was a 1:1 mixture of Ham’s F12 Medium and Dulbecco’s Modified Eagle’s Medium (DMEM/F12, Sigma, St. Louis, MO), with 2.5 mM l -glutamine (without phenol red). This base medium was supplemented with 10% fetal bovine serum (HyClone, Logan, UT) and 0.3 mg/mL G418 (Sigma, St. Louis, MO).
All scaffolds were sterilized by autoclaving at 121 °C for 30 min and then placed in 24-well plates. Cells were then seeded onto the samples. Cultures were maintained at 34 °C under a 5% CO 2 atmosphere as recommended by ATCC for hFOB 1.19. The medium was changed every 2–3 days for the duration of the experiment.
An MTT (3-(4,5-dimethylthiazol-2-yl)-2,5-diphenyl tetrazolium bromide) assay was used to evaluate cell proliferation. The MTT (Sigma, St. Louis, MO) solution of 5 mg/mL was prepared by dissolving MTT in PBS and the solution then filter-sterilized (0.2 μm). The MTT was diluted (100 μL into 900 μL) in DMEM/F12 medium and 1 mL of the diluted solution was then added to each of the samples. After 2 h of incubation, 1 mL of solubilization solution made up of 10% Triton X-100, 0.1 N HCl, and isopropanol was added to dissolve the formazan crystals. 100 μL of the resulting supernatant was transferred into a 96-well plate, and read by a plate reader at 570 nm. Cultures were evaluated at 3, 7 and 11 days. Data was normalized to pure TCP control scaffolds with corresponding pore parameters.
Samples for SEM observation were collected at days 3, 7 and 11 and fixed with 2% paraformaldehyde/2% glutaraldehyde in 0.1 M cacodylate buffer overnight at 4 °C. Post-fixation was performed with 2% osmium tetroxide (OsO4) for 2 h at room temperature. Fixed samples were then dehydrated in an ethanol series (30%, 50%, 70%, 95% and 100% three times), followed by a hexamethyldisilane (HMDS) drying procedure. After gold sputtering, samples were observed under FESEM.
2
Materials and methods
2.1
Fabrication of porous scaffolds
High purity oxide based sintering additives, silicon dioxide (SiO 2 ) (99%+ purity) and zinc oxide (ZnO) (99.9%+ purity) were purchased from Fisher Scientific (Fair Lawn, NJ). Synthetic β-tricalcium phosphate powder was obtained from Berkley Advanced Biomaterials Inc. (Berkeley, CA) with an average particle size of 550 nm and specific average surface area of 10–50 m 2 g −1 . Powder was made in batches of 100 g and doped with 0.25 wt% ZnO and 0.5 wt% SiO 2 as a binary dopant system. Dopant amounts were chosen based on previous research from our group . Powder was added to and mixed in 500 mL polypropylene Nalgene bottles, with 300 g of 5 mm diameter zirconia milling media. 150 mL of ethanol was added and ball milling was carried out for 6 h at 70 rpm to minimize the formation of agglomerates and increase the homogeneity of the powder. After milling, the Nalgene bottle was placed in an oven at 60 °C for 24 h for drying. Milling media were removed and the powder was further dried at 60 °C for another 24 h. Finally, agglomerates were removed using a mortar and pestle.
Cylindrical scaffold CAD (Computer Aided Design) files (diameter 7 mm and height 10.5 mm) were created with interconnected square channels of 1000 μm, 750 μm, and 500 μm sides ( Fig. 1 (a) ). Scaffolds were fabricated using a 3-D printer (R-1 R&D printer by ProMetal). The printing system consists of a deposition bed that starts with only a small base layer of powder, a feed bed that is filled with powder, a powder spreader, an ink jet print head and a drying unit. A schematic representation of operation is given in Fig. 1 (b). The printer head sprays binder onto the loose powder in the deposition bed according to the CAD pattern, the deposition bed is lowered by 20 μm and the feeder bed is raised by 60 μm. The spreader will then push the excess powder from the feeder bed and evenly spread it onto the lowered deposition bed. The process is repeated, layer by layer, until the CAD image is completely printed. Parts were built in 20 μm layers thickness with commercial organic water based binder obtained from ProMetal (Irwin, PA). Once finished, parts were dried at 150 °C for 1.5 h. Green scaffolds were then gently brushed clean and the remaining powder was removed by using a compressed air blower. After cleaning, green scaffolds were sintered in a muffle furnace at 1250 °C for 2 h. Important fabrication parameters that were optimized include binder selection, binder drop size and amount deposited, powder particle size, drying time between layers, powder spread rate, build layer thickness and powder feed to build ratio.
2.2
Microstructure, phase analysis and mechanical properties
The surface morphologies of the sintered scaffolds were observed under a field emission scanning electron microscope (FESEM) (FEI Inc., OR, USA). Phase analysis of sintered β-TCP samples with and without dopants was carried out by X-ray diffraction (XRD) using a Siemens D-500 X-ray powder Diffractometer (Siemens AG, Karlsruhe, Germany) with CuK α radiation and a Ni-filter. Each run was performed with 2 values between 10° and 60° at a step size of 0.02° and a count time of 0.5 s per step. Density was measured using Archimedes method. Samples were weighed initially dry and then submerged in boiling water for 3 min to remove any excess air that may be trapped in the porous structure. The samples were then transferred from the boiling water to room temperature water, where the weight was recorded again ( n = 3). Compressive strengths of undoped and doped TCP scaffolds were determined using a screw-driven universal testing machine (AG-IS, Shimadzu, Japan) with a constant crosshead speed of 0.33 mm/min. Compressive strength was calculated using the maximum load recorded and the sample dimensions. Compressive strength was tested on ten samples for each composition.
2.3
In vitro osteoblast cell–material interactions
Pure and doped TCP samples were studied for their cell–materials interactions using established human fetal osteoblast (hFOB) cells (hFOB 1.19, ATCC, Manassas, VA). The base medium for this cell line was a 1:1 mixture of Ham’s F12 Medium and Dulbecco’s Modified Eagle’s Medium (DMEM/F12, Sigma, St. Louis, MO), with 2.5 mM l -glutamine (without phenol red). This base medium was supplemented with 10% fetal bovine serum (HyClone, Logan, UT) and 0.3 mg/mL G418 (Sigma, St. Louis, MO).
All scaffolds were sterilized by autoclaving at 121 °C for 30 min and then placed in 24-well plates. Cells were then seeded onto the samples. Cultures were maintained at 34 °C under a 5% CO 2 atmosphere as recommended by ATCC for hFOB 1.19. The medium was changed every 2–3 days for the duration of the experiment.
An MTT (3-(4,5-dimethylthiazol-2-yl)-2,5-diphenyl tetrazolium bromide) assay was used to evaluate cell proliferation. The MTT (Sigma, St. Louis, MO) solution of 5 mg/mL was prepared by dissolving MTT in PBS and the solution then filter-sterilized (0.2 μm). The MTT was diluted (100 μL into 900 μL) in DMEM/F12 medium and 1 mL of the diluted solution was then added to each of the samples. After 2 h of incubation, 1 mL of solubilization solution made up of 10% Triton X-100, 0.1 N HCl, and isopropanol was added to dissolve the formazan crystals. 100 μL of the resulting supernatant was transferred into a 96-well plate, and read by a plate reader at 570 nm. Cultures were evaluated at 3, 7 and 11 days. Data was normalized to pure TCP control scaffolds with corresponding pore parameters.
Samples for SEM observation were collected at days 3, 7 and 11 and fixed with 2% paraformaldehyde/2% glutaraldehyde in 0.1 M cacodylate buffer overnight at 4 °C. Post-fixation was performed with 2% osmium tetroxide (OsO4) for 2 h at room temperature. Fixed samples were then dehydrated in an ethanol series (30%, 50%, 70%, 95% and 100% three times), followed by a hexamethyldisilane (HMDS) drying procedure. After gold sputtering, samples were observed under FESEM.
3
Results
3.1
Scaffold fabrication
The Ex One R1 3D printer was commercially designed and optimized for the use of metal powders. Our goal in this study was to produce scaffolds using CaP ceramic powders. One of the major challenges was the optimization of processing parameters to effectively produce these scaffolds using commercially available binder. Our process optimization research approach was first focused on how to produce a green TCP part followed by how to improve green strength of the part for mechanical handleability and depowderization to produce a 3D interconnected porous scaffold. Processing parameters used are given in Table 1 . The drop volume is a fixed parameter that is measured by the printer and is dictated by the viscosity and density of the binder. It is a measurement of the volume of each drop of binder that is released from a nozzle on the print head. The drop volume measured for the commercial binder used was 76 pL. The saturation percentage is based on the drop volume, packing efficiency of the powder layer and binder viscosity. The 100% value is a good starting point to work with because it is essentially a calculated estimate of how the binder will spread through the powder. If the saturation is too high, the binder will bleed into the surrounding powder, while too little saturation will result in extremely weak green scaffolds due to poor bonding between layers. The values that worked best were 110% for pure TCP powder and 100% for doped TCP powder. The pure TCP needed a little extra binder to maintain strength while handling and depowdering. The number of passes that the print head used to reach the desired saturation was 7 at a speed of 140 mm/s. The layer thickness was an important parameter because it dictated the depth resolution of the scaffold. The primary concern that was taken into consideration when choosing a layer thickness was the size of the powder particles. By using very fine powder, 550 nm, layer thicknesses of 20 μm for pure TCP powder and 30 μm for doped TCP powder were achieved while maintaining very smooth spreading. The doped powder had less depth resolution due to slight agglomeration of particles during the ball milling process. The feed powder to layer thickness ratio chosen was 3. This means that for every 20 or 30 μm that the build bed is lowered, the feed bed is raised by 60 or 90 μm, respectively. To help ensure that the powder is spread smoothly onto the build bed, a spread speed of 0.5 mm/s was used. This is the speed that the beds pass under the rolling spreader. As the beds move, loose powder from the raised feed layer is spread evenly onto the lowered build layer. By understanding the fixed processing parameters and using this information to adjust the variable processing parameters, we were able to successfully produce high resolution scaffolds with both doped and pure TCP powders.
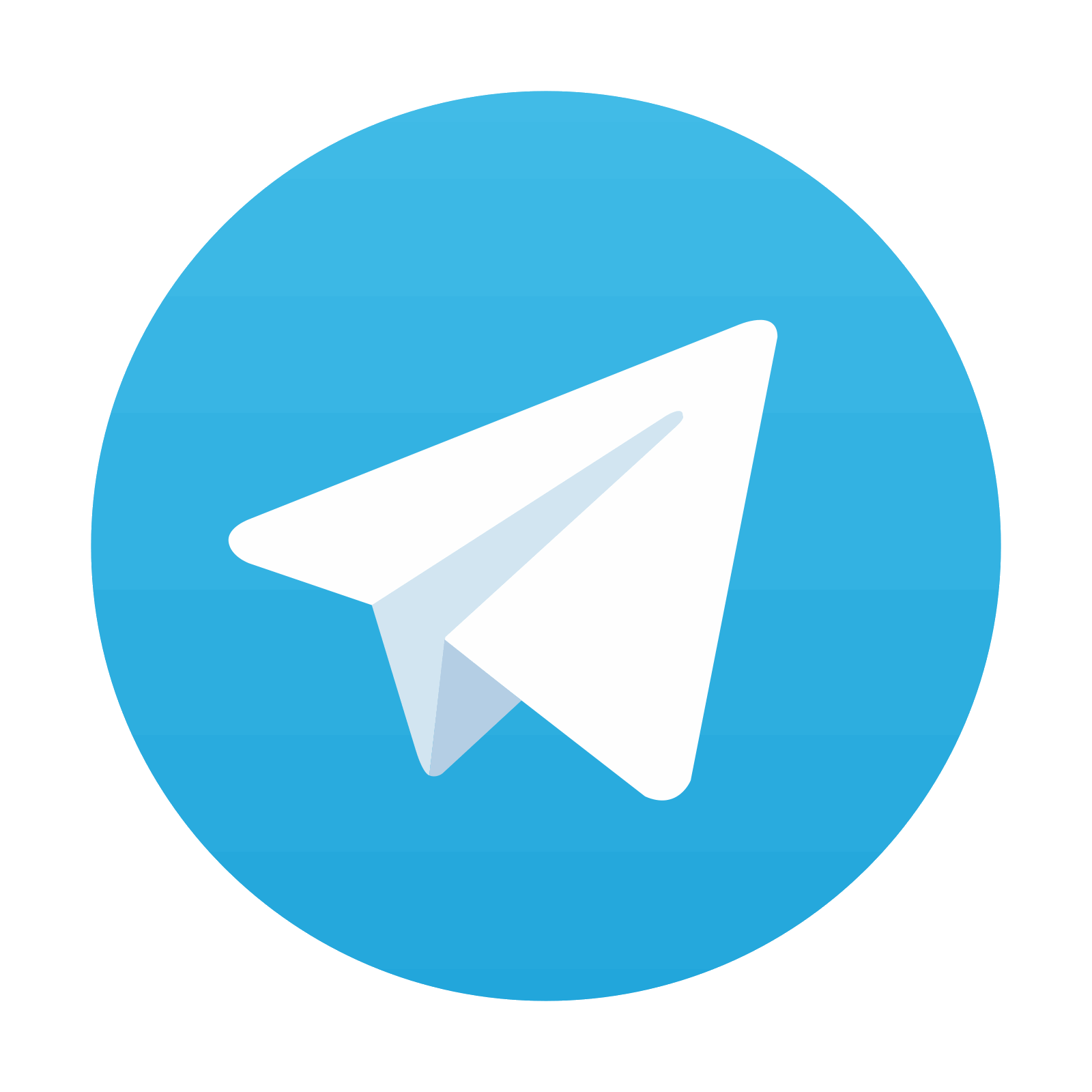
Stay updated, free dental videos. Join our Telegram channel

VIDEdental - Online dental courses
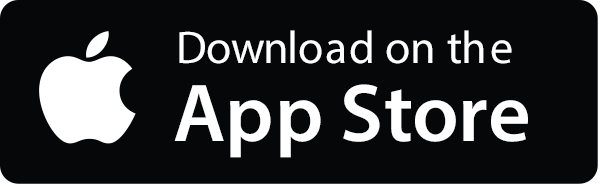
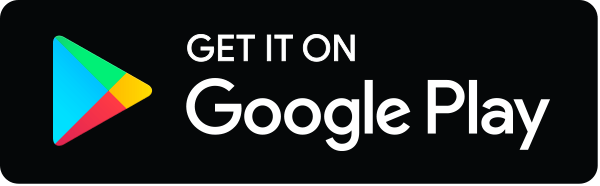
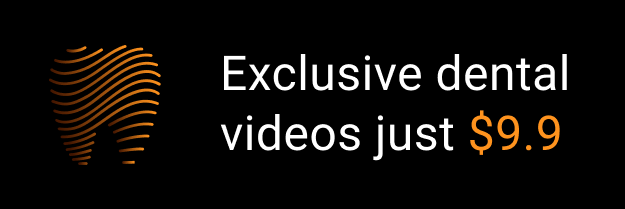