Abstract
Objective
To evaluate the influence of the addition of functionalized and non-functionalized TiO 2 nanostructures on properties of a resin composite.
Methods
TiO 2 nanostructures were synthesized and functionalized, using 3-(aminopropyl)triethoxysilane (APTMS) and 3-(trimethoxysilyl)propyl methacrylate (TSMPM). Characterizations were performed with XRD, EDS, TEM, and TGA. Resin composites containing Bis-GMA/TEGDMA, CQ, DABE, and barium-aluminum silicate glass were produced according to TiO 2 nanostructure (nanotube or nanoparticle), concentration (0.3 or 0.9 wt%), and functionalization (APTMS or TSMPM). The resin composite without nanostructures was used as control. The amount of fillers was kept constant at 78.3 wt% for all materials. The degree of conversion (DC – at 0 h and 24 h), maximum polymerization rate (Rp max ), and Knoop microhardness (KHN before and after ethanol softening) were evaluated. Data were analyzed with two-way ANOVA with repeated measures and Tukey’s HSD (α = 0.05).
Results
TGA results demonstrated that functionalizations were effective for both nanostructures. For DC, resin composites, time and interaction effect were significant (p < 0.001). Higher DC was found for 0.3-wt%-functionalized-nanotubes at 24 h. For nanoparticles, only 0.9-wt%-non-functionalized and 0.3-wt%-APTMS-functionalized showed DC similar to the control and all other groups showed higher DC (p < 0.05). Rp max was higher for 0.3-wt%-APTMS-nanotubes, which corresponded to higher DC after 24 h. The lowest Rp max occurred for 0.9-wt%-TSMPM-nanotubes, which showed smaller DC at 0 h. For KHN, resin composites, ethanol softening and interaction effect were significant (p < 0.001). KHN decreased after ethanol softening all groups, except for 0.3-wt%-TSMPM-nanotubes, 0.9-wt%-TSMPM-nanotubes, and 0.3-wt%-non-functionalized-nanoparticles.
Conclusion
The resin with 0.3-wt%-TSMPM-nanotubes showed higher DC after 24 h, while being the most stable material after the ethanol softening.
Significance
The addition of functionalized TiO 2 nanostructures in resin-based materials may improve the properties of the material.
1
Introduction
While resin-based materials are currently the most used in restorative dentistry, the mechanical properties still must be improved, especially when high stress-bearing occlusal areas, such as large cavities with lost cusps and incisal edges in patients with parafunctional habits are restored. For this reason, studies have been conducted to modify the structure of the material with the addition of nanostructures such as nanoparticles, nanotubes and nanofibers for reinforcement, resulting in an improvement on degree of conversion (DC) and mechanical properties [ ].
The study of different fillers is not new and it is well known that the type and quantity of filler particles added to resin composites might influence the wear resistance, shrinkage strain and stress, and DC [ , ]. On the other hand, more recently nanoscale structures such as titanium dioxide, silver, zinc oxide, silicon dioxide, and magnesium oxide have been used as fillers for reinforcing resin-based materials and improving biological properties [ ]. With the reduction of their size to nanoscale, particles increase their surface area per unit of volume and changes on physical and chemical properties of the material occur [ ]. Since surface atoms are not bonded to the maximum number of nearest neighbors and are in higher energy state than atoms at interior positions, as the particle size decreases, the surface phenomena begins to dominate [ ]. Additionally, quantum effects begin to appear for extremely small particles [ ].
Among nanostructures that may be used as additives for resin composites, the ones made of titanium dioxide (TiO 2 ) are interesting as they are nontoxic, inexpensive, and have high elastic modulus (230 GPa) and high refractive index [ ]. While mechanical properties may be improved by the incorporation of TiO 2 on resin-based materials [ ], the high refractive index is interesting as not only the degree of opacity, but the brightness and opalescence could be modulated by the addition of TiO 2 [ ].
One of the most important characteristics of TiO 2 nanostructures is the photocatalytic property [ , , , ]. This phenomenon occurs when TiO 2 is exposed to light irradiation that has higher energy than its band gap. Photons are absorbed and an electron is promoted to the conduction band, leaving a hole in the valence band [ ]. The excited electrons in the conduction band facilitate reduction of electron acceptors, while holes in the valence band facilitate oxidation of electron donors [ ]. Additionally, it has been demonstrated that the hole created in the valence band is able to initiate radical polymerizations of various monomers such as methyl methacrylate [ ]. Thus, the photocatalytic property may be interesting to improve the polymerization of resin composites, especially the ones who employ the conventional CQ/amine system, as the free electrons generated from the photocatalytic reaction of TiO 2 could change how the electron-donor amine reacts with CQ.
It is known that different morphologies of TiO 2 nanostructures have different behaviors [ , ]. The TiO 2 nanotube is a one-dimensional (1D) hollow structure that promotes interaction with the medium, due its large internal and external surface area [ , , ]. More hydroxyl groups are present on its surface when compared to other morphologies [ ] and its shape favors the conductivity of electrons [ ]. The TiO 2 nanoparticle, on the other hand, is a zero-dimensional (0D) structure that has a spherical shape and large specific surface area [ , , , ]. Given that the photoreactions occur on the surface of the photocatalyst, it is hypothesized that the difference in morphology between TiO 2 nanotubes and nanoparticles associated with their different electronic potential will influence their performances when added to a resin matrix.
Though TiO 2 nanostructures may be used to improve the properties of methacrylate resins [ , ], at the same time, they tend to agglomerate in their native state [ ]. A way to overcome this disadvantage is the functionalization of the TiO 2 surface [ , , ]. This is possible because TiO 2 nanostructures are mainly terminated by hydroxyl groups (−OH), which can be functionalized by various organic bifunctional molecules, such as silanes, carboxylic acids, esters, acid chlorides, and carboxylate salts [ ]. The functionalization process allows the nanostructures to remain chemically stable in uniform sizes and is an alternative to overcome the effects of the agglomeration, due to increase in the dispersion of nanostructures throughout the resin matrix [ , , , ].
The organosilane (3-aminopropyl)trimethoxysilane (APTMS) is a bifunctional molecule that contains a silane functional group and a amine ground on the other end [ , , ]. The silane has higher affinity for the substrate and interacts covalently with hydroxyls (−OH) groups present on the surface of TiO 2 nanostructures to form Si-O-Ti bonds, while the other terminal amine functional group stays free to interact with other molecules [ ]. The functionalized TiO 2 surfaces by APTMS are uniform multilayers characterized by a dense deposition that completely covers the surface [ ]. Another coupling compound used in resin composites that has the function of interconnecting organic and inorganic components is 3-(trimethoxysilyl)propyl methacrylate (TSMPM) [ ]. These compounds create strong linkages between nanostructures and the matrix [ ] and become an excellent feature for the formation of new resin-based materials.
Therefore, the aim of this study was to evaluate the influence of the addition of different concentrations of functionalized and non-functionalized TiO 2 nanostructures on the properties of an experimental resin composite. To this end, this study was divided into two stages: 1) synthesis of TiO 2 nanoparticles and nanotubes, functionalization with APTMS or TSMPM, and characterization of the nanostructures, and 2) addition of the nanostructures to experimental resin composites for analyses of DC immediately and 24 h after light activation, polymerization kinetics, and Knoop microhardness before and after softening in ethanol. The null hypotheses were that the addition of different TiO 2 nanostructures (nanotubes or nanoparticles), TiO 2 nanostructures concentrations (0.3 wt% or 0.9 wt%), and functionalizations made on nanostructures (APTMS or TSMPM) would not result in differences in the: 1) DC, 2) polymerization kinetics, 3) Knoop microhardness, 4) Knoop microhardness after softening in ethanol, and 5) percentual loss of hardness.
2
Materials and methods
2.1
Route for TiO 2 nanostructures preparation
An alkaline hydrothermal process was used to synthesize TiO 2 nanotubes [ ]. Twelve grams of anatase titanium dioxide (Sigma-Aldrich, Darmstadt, HE, Germany) were mixed with 120 mL of a 10 M solution of NaOH and kept for 24 h at 110 °C. The pasty substance formed at the canister bottom was mixed with deionized water and centrifuged for 10 min at 20,000 rpm. The supernatant was discarded, 0.1 M HCl was added, and the solution was again centrifuged. The process was repeated until reaching pH 4. The supernatant was then removed and the sample was dried in a muffle at 200 °C for 24 h at a heating/cooling rate of 10 °C/min.
A sol-gel route was used to synthetize TiO 2 nanoparticles [ ]. A mixture of 185.0 mL of distilled water, 56.7 mL of isopropanol (Merck & Co Inc, Darmstadt, HE, Germany) and 2.6 mL of nitric acid (Synth, Diadema, SP, Brazil) was added in an Erlenmeyer. Then, 15 mL of 99.9% anatase titanium isopropoxide (Sigma-Aldrich) was added under magnetic stirring for 30 min. In this step, an increase in the turbidity of the solution and the formation of white precipitates were observed, indicating that hydrolysis and condensation were occurring. Upon heating of the solution, a peptization process started. The reaction mixture was kept under magnetic stirring at 85 °C until evaporation of the solvent. The paste mixture was placed in a conventional oven to form the nanoparticles at 450 °C for 2 h with heating/cooling rate of 1 °C/min [ , ].
2.2
Functionalization of TiO 2 nanostructures
The hydroxyl groups present on the surface of TiO 2 nanostructures were functionalized using APTMS (Sigma-Aldrich, Darmstadt, HE, Germany) and TSMPM (Acros-Organics, Loughborough, United Kingdom) ( Fig. 1 A and 1B). The functionalization route was the same for both compounds. APTMS and TSMPM (2 mL) were added to the suspension of nanoparticles or nanotubes (0.5 g) in ethanol (50 mL). The reaction mixture was maintained at 50 °C under magnetic stirring for 24 h. After this period, the material was centrifuged and dried under vacuum for approximately 24 h [ ].

2.3
Characterization of TiO 2 nanostructures
X-ray diffraction (XRD) was performed (n = 3) using Cu K-alfa radiation (40 kV, 15 mA) in a Rigaku MiniFlex 600 diffractometer (Bruker AXS, Karlsruhe, BW, Germany) to determine the crystalline phase of TiO 2 nanostructures. XRD samples were prepared by gently grinding 0.1 g of the calcined TiO 2 nanostructures using a mortar and a pestle. The powder was then placed on the plastic flat plate of the XRD and positioned in the diffractometer. Data were collected at a scattering angle of 2° from 20° to 80° with 10°/min scan speed.
Elemental analysis was performed (n = 3) and the TiO 2 nanostructures set was mapped using energy-dispersive x-ray spectroscopy (EDS) analyses (Zeiss EVO-LS15, Jena, TH, Germany). Samples were coated with gold to make them electrically conductive and suitable for SEM.
The efficiency of the functionalization of TiO 2 nanostructures was verified using thermogravimetric analysis (TGA) (Discovery TGA55, TA Instruments – Waters LLC, New Castle, DE, USA). For this purpose, approximately 15 mg of filler was placed in a high temperature platinum pan and subjected to a heat ramp (from 50 °C to 850 °C, at 10 °C/min). Percent mass loss was recorded as a function of temperature and all samples were evaluated in triplicate. Results were reported as the average total mass loss (%).
The morphology of TiO 2 nanostructure powders was examined by transmission electron microscopy (TEM) (Phillips CM 200, Eindhoven, Netherlands). For TEM evaluation, samples were prepared in copper grids (TED PELLA, ultrathin C type A, 400 mesh). The sample was dispersed in isopropyl alcohol and kept in an ultrasonic bath for 10 min for redispersion. One milliliter was dripped onto the copper grid, which was dried at room temperature for the analysis.
2.4
Preparation of resin composites
Resin composites were prepared by mixing bisphenol A glycerolate dimethacrylate (BIS-GMA) and triethyleneglycol dimethacrylate (TEGDMA) at the mass ratio of 75/25%. To this mixture, 0.25 wt% of camphorquinone (CQ) and 1 wt% of ethyl 4-(dimethylamino) benzoate (DABE) were added. Barium-aluminum silicate glass of 1μ (Schott, Elmsford, NY, USA) silanized by the manufacturer with a methacrylate silane was added in amounts ranging from 77.4 to 78.3 wt%, depending on the amount of nanostructure added to the resin composite. The basic resin composite was modified by incorporating 0.3 wt% or 0.9 wt% of non-functionalized or functionalized TiO 2 nanostructures. For the sake of maintaining the same amount of filler in all groups, while adding nanostructures the equivalent percentage by weight of barium-aluminum silicate glass was removed from the basic resin composite as shown in Table 1 . The resin composite without nanostructures was used as a control. All materials were obtained from Sigma-Aldrich (Sigma-Aldrich, Darmstadt, HE, Germany) and all the composites were prepared by FGM (Joinville, SC, Brazil).
Description | Code | Filler (wt%) | TiO 2 (wt%) |
---|---|---|---|
Control | CTL | 78.3 | Zero |
TiO 2 nanotubes | T-0.3 | 78.0 | 0.3 |
T-0.9 | 77.4 | 0.9 | |
TiO 2 nanotubes + APTMS | T-A-0.3 | 78.0 | 0.3 |
T-A-0.9 | 77.4 | 0.9 | |
TiO 2 nanotubes + TSMPM | T-T-0.3 | 78.0 | 0.3 |
T-T-0.9 | 77.4 | 0.9 | |
TiO 2 nanoparticles | P-0.3 | 78.0 | 0.3 |
P-0.9 | 77.4 | 0.9 | |
TiO 2 nanoparticles + APTMS | P-A-0.3 | 78.0 | 0.3 |
P-A-0.9 | 77.4 | 0.9 | |
TiO 2 nanoparticles + TSMPM | P-T-0.3 | 78.0 | 0.3 |
P-T-0.9 | 77.4 | 0.9 |
2.5
Degree of conversion (DC) and maximum rate of polymerization (Rp max )
The DC was evaluated by FTIR (IR Prestige-21 Shimadzu, Tokyo, Japan), using an ATR attachment (MIRacle ATR, Pike Technologies, Madison, WI, USA) at 4 cm −1 resolution and 32 scans in the range of 4000−600 cm −1 (n = 5). The initial reading was performed by adding small portions of the non-polymerized material inside a disc-shaped (6 mm diameter, 1 mm thick) Teflon mold placed on top of the ART crystal. After the initial reading the resin composite was light activated for 20 s using a LED device (VALO, Ultradent Products, South Jordan, UT, USA) operating at 1000 mW/cm 2 . The irradiance was checked with a digital flat-response power-meter (New Port – model 2936-R, Irvine, CA, USA). Spectral bands were obtained and the wavelength peaks of 1636 cm −1 (aliphatic bonds, C C) and 1608 cm −1 (aromatic bonds) were identified for each sample. DC values were obtained immediately after light activation and 24 h after storage in dark canisters at 37 °C.
Where R is the ratio of the absorbance at 1636 cm- 1 and absorbance 1608 cm -1 , calculated for both cured and uncured resin composites.
The Rp max was obtained after real-time assessment of the polymerization kinetics in near-IR (n = 3). Disk samples (5 mm in diameter, 0.8 mm in thickness) were prepared in a rubber mold by sandwiching resin composites between two glass slides. Discs were placed in the chamber of an infra-red spectrometer (Nicolet 6700, ThermoScientific, Madison, WI, EUA) and irradiated with a mercury arc lamp (Acticure, EXFO Acticure 4000 UV Cure; Mississauga, ON, Canada) filtered to 320−500 nm for 180 s, delivering 1000 mW/cm 2 directly to the specimen. Spectra were collected in real-time during light activation, with 2 scans per spectrum at a resolution of 4 cm −1 . In this analysis, the DC was calculated in near-IR at 6165 cm −1 based on the area of the methacrylates vinyl overtone [ ]. The Rp max was the first derivative of DC with respect to time.
2.6
Knoop Microhardness (KHN) and softening in ethanol
The KHN evaluation was performed on the same resin disks used in the FTIR-ATR evaluation, after storage for seven days in dry dark canisters. Samples were placed in the microhardness tester (Buehler, Lake Bluff, IL, USA) and three indentations with a distance of 1 mm among them were performed under a 50 g load for 10 s.
After the initial microhardness evaluation, samples were immersed in 100% ethanol (Dinâmica, Indaiatuba, SP, Brazil) at room temperature for 24 h [ ]. After this period of softening in ethanol, microhardness was again evaluated using the same parameters described above. The percentual loss of hardness was obtained with the formula described below:
Percentual loss of hardness = [(1-(KHN ethanol / KHN))*100]
Where KHN ethanol is the Knoop microhardness number after softening in ethanol and KHN is the Knoop microhardness number before softening.
2.7
Statistical analyses
DC and KHN data were analyzed with two-way ANOVA with repeated measures and Tukey’s HSD, considering resin composites and storage time as independent variables for DC, and resin composites and softening in ethanol as independent variables for KHN. Rate of polymerization and percentual loss of hardness data were analyzed with one-way ANOVA and Tukey’s HSD. An overall significance level was set at α = 0.05. Statistical analyses were performed using Statistica 10 (Stat Soft Inc, Hamburg, Germany).
3
Results
3.1
Characterization of TiO 2 nanostructures
Fig. 2 shows TEM images demonstrating the morphology of TiO 2 nanostructures. TiO 2 nanotubes images confirmed the presence of hollow tubular structures with diameter of approximately 10 nm and length of approximately 200 nm. TiO 2 nanoparticles images showed a uniform morphology presented as nanospheres with diameter of approximately 10 nm.

The XRD analysis showed a prominent diffraction peak at 25°, in addition to other peaks at 38°, 48°, 54°, 56°, 63°, and 68° ( Fig. 3 ). These diffraction peaks represent the anatase crystal phase of TiO 2 .

Based on EDS analyses, Ti and O were the most abundant elements in non-functionalized TiO 2 nanostructures ( Fig. 4 ). In APTMS- ( Fig. 5 ) and TSMPM-functionalized groups ( Fig. 6 ), higher percentages of carbon (C), sodium (Na), aluminium (Al) and silicon (Si) were detected, suggesting the presence of bifunctional compounds used for functionalization.

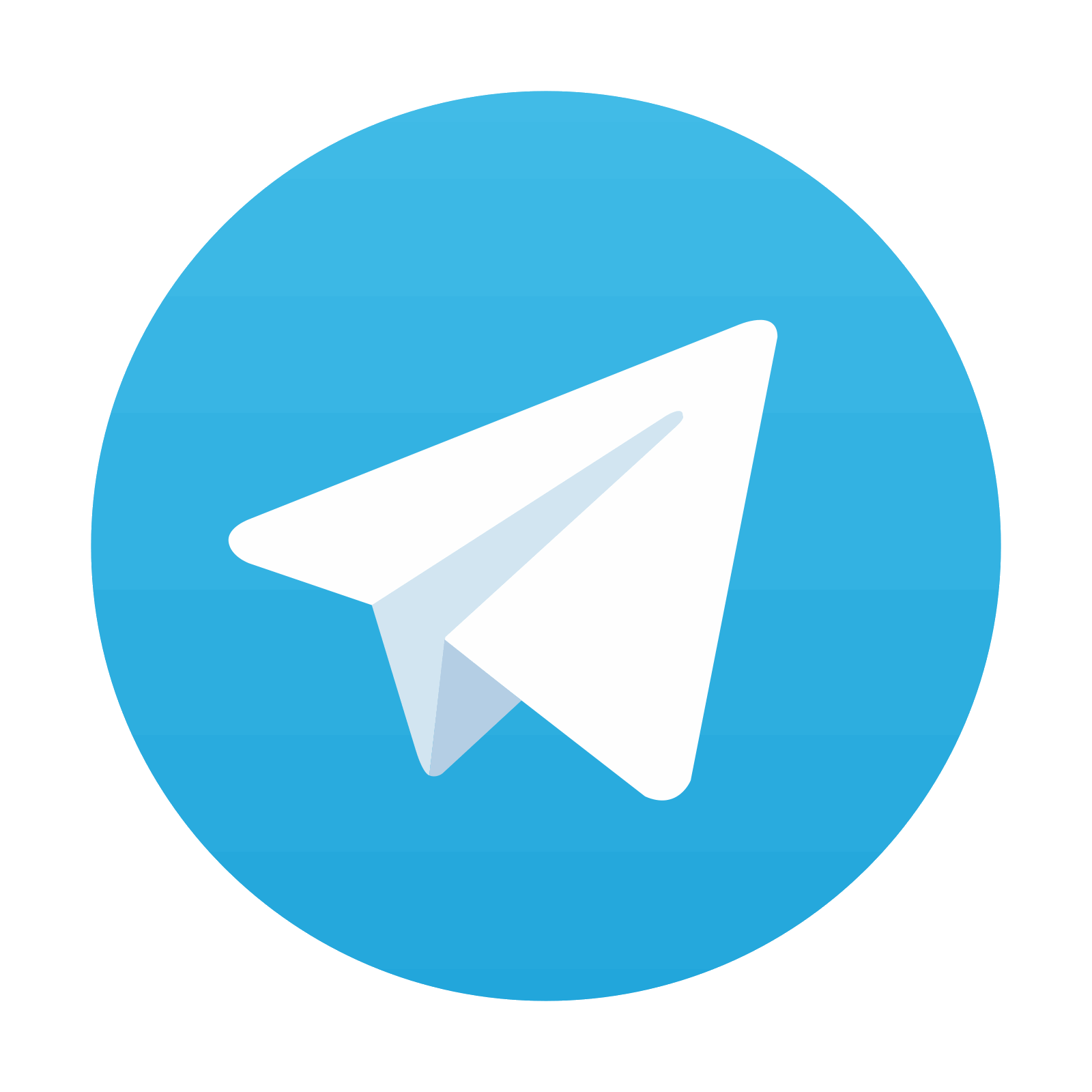
Stay updated, free dental videos. Join our Telegram channel

VIDEdental - Online dental courses
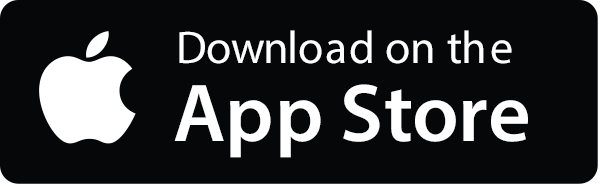
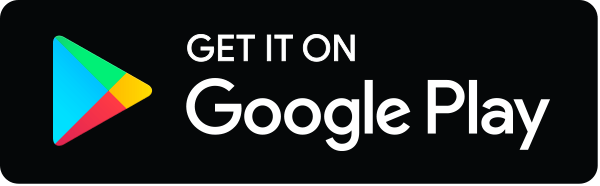
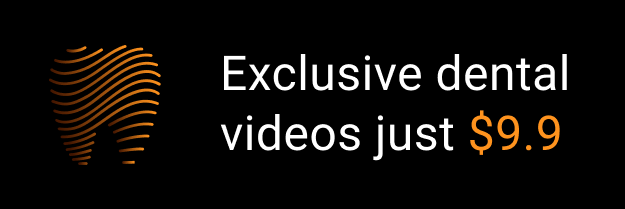