Highlights
- •
For the first time, dentin demineralization has been studied with different spectroscopic techniques.
- •
Results from different techniques were successfully correlated.
- •
Raman spectroscopy proved to be a good diagnostic tool for evaluation of dentin demineralization.
- •
The quantification algorithms can be easily calibrated for in vivo applications.
Abstract
Early detection of dental caries and variations in composition/structure of both enamel and dentin represents an important issue in modern dentistry. Demineralization has been associated to teeth discoloration, development of caries, and formation of cavities.
Objective
In this study, we systematically monitored the processes of demineralization/remineralization in dentin samples by means of three different spectroscopic techniques, namely, Raman spectroscopy, X-Ray Photo-electron spectroscopy (XPS), and X-Ray Diffractometry (XRD).
Methods
Bovine dentin samples were first exposed to acidic solutions and their structure systematically monitored as a function of time and pH. Then, the samples were rinsed in artificial saliva to simulate remineralization.
Results
The above three spectroscopic techniques provided quantitative structural information spanning from the nanometer to the millimeter scale of sample penetration depth. An irreversible level of demineralization was reached when dentin was exposed to pH 2 beyond a time threshold of 6 h, successive treatments with artificial saliva being unable to restore the mineral fraction. On the other hand, short-term treatments at pH 5 and long-term treatments at pH 6 could partially or completely recover the dentin structure within one week of remineralization treatment.
Significance
Two specific Raman parameters, namely, the bandwidth of the symmetric phosphate-stretching signal and the mineral-to-matrix intensity ratio, showed strong correlations with XPS and XRD data, and matched laser microscopy observations. Such correlations open the path to apply Raman spectroscopy in monitoring dentin demineralization in vivo and provide quantitative working algorithms for the prevention of oral caries.
1
Introduction
The connective tissue, or pulp, at the teeth core is composed of three areas: chamber, horns, and radicular canals. The pulp is surrounded by dentin to which it is intimately connected through a uniform distribution of dentin tubules. Due to the constant deposition of dentin by odontoblasts, the chamber tends to become smaller with age [ , ]. Dentin is also partially covered by the enamel structure, which in principle constitutes the only visible part of the tooth in people with healthy and well-developed gums [ ]. However, dentin could become directly exposed to oral environment in presence of gum-associated pathologies (gum recession) such as periodontitis [ ]. Dentin and enamel greatly differ in the amount of apatite mineral fraction embedded in the matrix of organic collagen (50% in dentin vs . 95% in enamel), and in their biological functions as well. Dentin is tougher than enamel thus providing mechanical support to enamel, but also allows the pulp to access to the external environment through the dentin tubules [ ]. However, upon exposure to oral environment, it responds to external stimuli of thermal, tactile, osmotic, chemical, and electrical nature resulting in hypersensitivity and pain [ ]. Dentin hypersensitivity is commonly treated by sealing the tubules with lasers or adhesive sealers, or by nerve desensitization. Dentin can also become exposed to the oral environment due to cavities or acidic erosion through enamel [ ]. This is often referred to as the third stage of tooth decay and can be detected optically as the teeth locally change in color [ ]. While monitoring the occurrence of both cavities and gums recession is key in the prevention of oral diseases, a non-intrusive diagnostic tool for assessing dentin quantity and quality [ ] could find practical applications in dentistry.
This study follows previous reports about the use of spectroscopic techniques and, in particular, Raman spectroscopy, for monitoring early demineralization in enamel [ ].
In previous published researches we were able to correlate the width of the bands related to phosphate vibrations (at about 960 cm −1 ) to the levels of demineralization of enamel after exposure to acidic environments [ ], but we could not give a quantification due to the absence of secondary bands related to amides. Preliminary qualitative demineralization analyses could be performed on osteoporotic bone samples, due to the relatively high content of collagen [ ], but unlike teeth bone [ ] has a wide degree of variability in composition and orientation, which affects the reliability (and representatively) of the spectroscopic results. More sophisticated algorithms, focused on light polarization and crystal orientation, could reveal differences in polarization anisotropy between early caries and sound enamel [ ], but this approach, even if predictive and accurate, is more time consuming, requiring both huge quantities of spectroscopic data and long post-processing analyses.
Different from those studies, this research focuses on the dentin structure and on the balance between mineral and matrix fractions. Spectroscopic techniques are already in use for the characterization of teeth [ , ], but they generally require large equipment and are hardly applicable in vivo . One exception is represented by fluorescence-based handheld devices [ ], already appeared in the marked at the end of last century [ ]. However, because of the physical nature of the fluorescence signal, those devices are unable to provide any quantitative chemical or crystallographic information of dental tissue. Conversely, the Raman spectrum is intrinsically linked to the structure of tissue. However, in order to make the Raman spectroscopic outputs fully quantitative, specific calibrations are needed.
Enamel and dentin are constantly subject to demineralization and remineralization processes in vivo [ ], but while many factors can be responsible for demineralization, such as food intake [ ], presence of microbes [ ], excessive brushing [ ], dry mouth [ ], bruxism [ ], only proper salivation and dental hygiene [ ] can compensate the dissolution by re-introducing Calcium and Phosphate ions, in a slightly alkaline environment. Moreover, the dynamics of the demineralization/remineralization processes are complex and difficult to predict clinically [ ], but once a certain threshold has been reached, demineralization might become irreversible [ ].
Nowadays, remineralization can be stimulated using specific products, in particular calcium phosphate solutions [ ], but while these solutions can initially boost the amount of mineral apatite, there is still little scientific evidence about their long term beneficial effects [ ].
In this paper, we performed demineralization/remineralization calibrations for dentin by matching Raman measurements with two quantitative spectroscopic techniques, namely XPS and XRD, and substantiating the findings according to laser microscopy observations. We found clear correlations between the Raman results and the outputs of other techniques, which fully validate the Raman approach. Raman spectrometers have nowadays been miniaturized to pocket portable devices and can be converted to remote control by the use of optical fibers. Hence, the availability of solid correlations with well-established spectroscopic techniques makes the Raman approach a quantitative tool for dental diagnostics that fits the preventive management of oral care required in modern dentistry.
2
Materials and methods
2.1
Sample preparation and treatments
An explanatory draft of dentine sample preparation and probe/sample interaction during spectroscopic assessments is presented in Fig. 1 . Bovine teeth samples were cut into mm-sized cubes from areas just below the region covered by enamel. Their surface was lightly polished to obtain flat surfaces. The surface morphology of the samples was analyzed using a confocal scanning laser microscope (Laser Microscope 3D and Profile measurements, Keyence, VKx200 Series, Osaka, Japan). All images were collected at various magnifications ranging from 10× to 150×. Roughness values were measured on 10 randomized 100 × 100 μm square areas per sample. The size of the dentin tubules was quantitatively assessed using ImageJ [ ] on post-processed images. Optical distortions due to the orientation of the tubules were automatically corrected by software.

Groups of 6 samples were immersed in acidic to neutral solutions at pH 2, 5, 6, and 7 (ultra-pure deionized water) for increasing intervals of time spanning from 6 to 24 h. For the acidic solutions, glacial acetic acid has been diluted in demineralized water in order to obtained the desired pH. After demineralization, half of the samples were immersed in artificial saliva (1.5 mmol/l CaCl 2 , 0.9 mmol/l KH 2 PO 4 , 130 mmol/l KCl, 20 mmol/l HEPES buffer solution) for one week in order to promote remineralization. The size of dentine tubules was quantitatively assessed by laser microscopy in pristine, demineralized, and remineralized samples as a function of time and pH ( cf . Fig. 1 ).
2.2
Sample characterizations
Raman spectra were acquired using a confocal (optical) microprobe at room temperature by means of a single monochromator (T-64000, Jobin-Yvon/Horiba Group, Kyoto, Japan) equipped with a nitrogen-cooled 1024 × 256 pixels CCD camera (CCD-3500V, Horiba Ltd., Kyoto, Japan). The excitation frequency used in the experiment was a 514 nm blue line of an Ar-ion laser operating at a power of 80 mW. Under these experimental conditions, the penetration depth of the laser (Raman probe size) was ∼10 μm ( cf . Fig. 1 ). The spectrum integration time was typically 15 s with each recorded spectra being averaged over three successive measurements. All spectra were acquired on multiple windows, ranging from 400 to 1750 cm −1 . A total number of 81 spectra were acquired on each of the studied sample. Raman data were analyzed by commercially available software (LabSpec, Horiba/Jobin Yvon, Kyoto, Japan). Raman band parameters were obtained by fitting the raw experimental spectra with mixed Lorentzian–Gaussian curves.
XPS experiments were carried out using a photoelectron spectrometer (JPS-9010 MC; JEOL Ltd., Tokyo, Japan) with an X-ray source of monochromatic MgK α (output 10 kV, 10 mA). The measurements were conducted in a vacuum chamber at around 1.5 × 10 7 Pa, upon setting the analyzer pass energy to 10 eV and the voltage step size to 0.1 eV. For all samples, a wide scan was performed before acquiring 10 scan repetitions on each investigated region and specifically: Carbon (C1s, ∼285 eV), Oxygen (O1s, ∼530 eV), Nitrogen (N1s, ∼400 eV), Calcium (Ca2p, ∼345 eV) and Phosphorous (P2p, ∼130 eV). The X-ray angle of incidence and the takeoff angle were 34° and 90°, respectively. Measurements were repeated on 3 different areas for each sample ( n = 3). The penetration depth of the XPS probe was in the order of 10 nm ( cf . Fig. 1 ).
XRD analyses were performed on a Rigaku Miniflex 600 system (Rigaku Corporation, Tokyo, Japan), using the CuK α radiation (40 kV and 15 mA). Diffraction spectra were acquired in the range 10°–80° with a step size of 0.0158 at a rate of 1.5 s/step. The penetration depth of the XRD probe was in the order of 1 mm ( cf . Fig. 1 ). Raw data were treated with searching for peaks and determining background. The resulting patterns were then matched with ICCD/ICSD database reference patterns after applying elemental database restrictions. The database search provided a list of reference patterns with a match score, indicating each pattern’s overlap with peak intensity and position in the experimental spectrum. The phases were matched based on scoring from the software, reference pattern quality, and qualitative analysis.
2.3
Statistical analysis
Data has been presented as mean value ± one standard deviation. One-way ANOVA has been used for the statistical analysis of histograms, while the R 2 value has been used to estimate the reliability of fitting equations on x–y plots. The p < 0.05 was considered statistically significant, and labeled with an asterisk, while p < 0.02 was marked with two asterisks to reflect the higher reliability. Non statistically significant results were marked with n.s . or left unmarked.
3
Experimental results
3.1
Morphological characterization of the dentin surface
Fig. 2 shows laser microscopy images of the pristine dentin surface (a) and those of samples treated at pH 2 and pH 5 ((b) and (c), respectively). The same samples after remineralization are given in (d) and (e), respectively. Demineralization in acidic environment at both pH values (red arrows) resulted in an increase of the average size of dentin tubules. The increased diameter of the tubules was clearly a consequence of mineral dissolution in acidic environment, as the amount of dissolved material was a function of both pH and immersion time, with pH 2 being a more aggressive environment than pH 5. For exposures of any period of time at pH 6, any material dissolution resulted to be hardly detectable.

After remineralization ( Fig. 2 (d) and (f)), the diameter of the tubules decreased, but the surface morphology appeared more rough and irregular, due to the presence of preferential locations for mineral deposition.
Fig. 3 shows the average diameters of the dentin tubules after up to 24 h of immersion in three different environments at pH 2, 5 and 6, as compared to the untreated average value. As seen, the treatment at pH 6 has little (if any) influence on the dentin tubule diameters. Moreover, the results of this group of samples are scattered mainly because of a lack of uniformity between different specimens. On the other hand, after both treatments at pH 2 and 5, the diameter of the dentin tubules showed a clearly detectable increase after 6 h of immersion. The noticeable decrease in diameter after 24 h at pH 2 is an effect of the distorted morphology of dentin tubules ( cf . Fig. 2 (c)). A few tubules are almost completely occluded by peritubular dentin [ ], which reduces the apparent size of the pores.

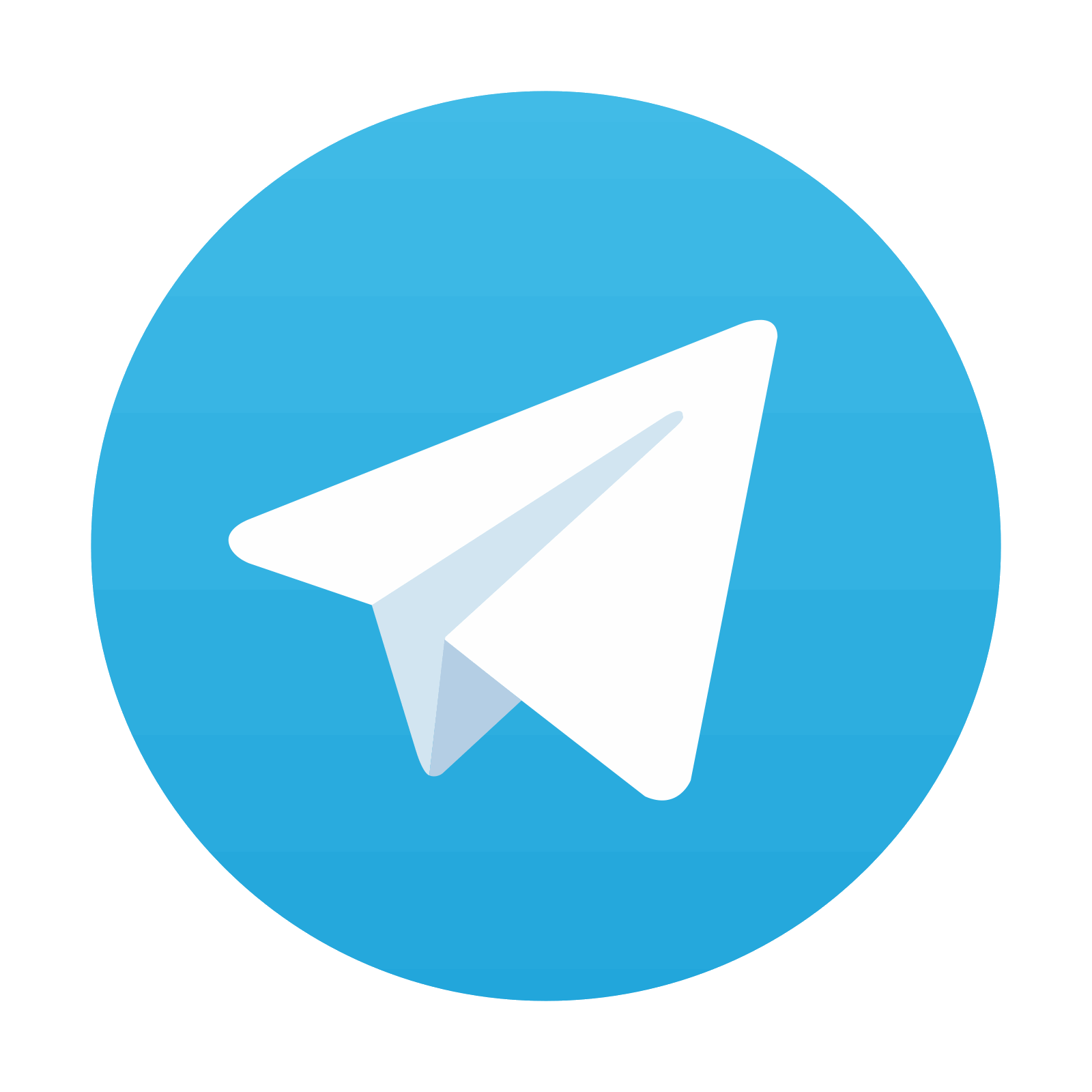
Stay updated, free dental videos. Join our Telegram channel

VIDEdental - Online dental courses
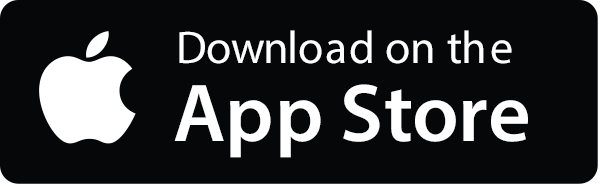
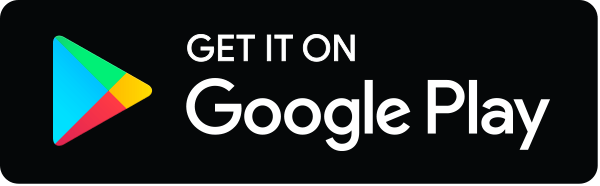
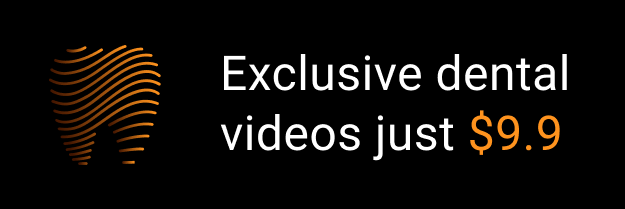