Introduction
Our objective was to investigate the effect of low-frequency mechanical vibration (LFMV) on the rate of tooth movement, bone volume fraction, tissue density, and the integrity of the periodontal ligament. Our null hypothesis was that there would be no difference in the amount of tooth movement between different values of LFMV.
Methods
Sixty-four male CD1 mice, 12 weeks old, were used for orthodontic tooth movement. The mice were randomly divided into 2 groups: control groups (baseline; no spring + 5 Hz; no spring + 10 Hz; and no spring + 20 Hz) and experimental groups (spring + no vibration; spring + 5 Hz; spring + 10 Hz; and spring + 20 Hz). In the experimental groups, the first molars were moved mesially for 2 weeks using nickel-titanium coil springs delivering 10 g of force. In the control and experimental groups, LFMV was applied at 5, 10, or 20 Hz. Microfocus x-ray computed tomography analysis was used for tooth movement measurements, bone volume fraction, and tissue density. Additionally, immunostaining for sclerostin, tartrate-resistant acid phosphatase (TRAP) staining, and picrosirius red staining were used on the histologic sections. Simple descriptive statistics were used to summarize the data. Kruskal-Wallis tests were used to compare the outcomes across treatment groups.
Results
LFMV did not increase the rate of orthodontic tooth movement. Microfocus x-ray computed tomography analysis showed increases in bone volume fractions and tissue densities with applications of LFMV. Sclerostin expression was decreased with 10 and 20 Hz vibrations in both the control and experimental groups. Additionally, the picrosirius staining showed that LFMV helped in maintaining the thickness and integrity of collagen fibers in the periodontal ligament.
Conclusions
There was no significant increase in tooth movement by applying LFMV when compared with the control groups (spring + no vibration).
Highlights
- •
We studied low-frequency mechanical vibration (LFMV) on tooth movement and bone.
- •
The rates of tooth movement did not differ between the experimental groups.
- •
LFMV did not increase the rate of orthodontic tooth movement.
- •
Bone volume fraction and tissue density were higher in the LFMV groups.
- •
LFMV at 5, 10, and 20 Hz had no deleterious effects on the periodontal ligament.
Tooth movement involves both remodeling and modeling of bone. Remodeling and modeling involve a coordinated action of osteoclasts and osteoblasts in response to mechanical loading. Moreover, inflammatory mediators (interleukin [IL]-1, IL-2, IL-6, IL-8, and tumor necrosis factor-alpha) are released after mechanical stimulus or injury, triggering the biologic process associated with orthodontic tooth movement (OTM).
Currently, orthodontic treatment requires approximately 24 to 30 months of intervention to complete the treatment. The longer duration of treatment is a great concern and poses high risks for caries, root resorption, and decreased patient compliance and satisfaction. Thus, accelerating OTM and shortening the total treatment duration is a primary goal of the orthodontist, and it can prevent detrimental effects of longer treatment time and increase patient satisfaction.
Nishimura et al have shown that the application of cyclical forces (60 Hz) on the maxillary first molar increases the rate of OTM. However, the main drawback of the Nishimura study was the method of force application (transpalatal expansion spring). The force was applied to accelerate tooth movement in the first order (buccolingually) rather than in the second order (mesiodistally), which comprises the majority of OTM. Moreover, it may confound the actual tooth movement because of its skeletal effects. Studies have shown that whole body vibration (30, 45, and 90 Hz) may have an anabolic response on bone mass and architecture. Miles et al, in their randomized controlled trial, showed that application of 111 Hz of vibrational frequency for 20 minutes per day did not speed tooth movement when compared with the controls. Kalajzic et al showed the inhibitory effect of cyclical forces (30 Hz and 40 g of force applied with an electromechanical actuator) on OTM in rats; moreover, they showed the deleterious effects of the cyclical forces on the periodontal ligament (PDL). The major drawback of their model was higher cyclical force (40 g). The effect and mechanism of low-frequency mechanical vibrations (LFMV) (≤20 Hz) on OTM and paradental tissues still remain unclear. To our understanding, this is the first in-vivo study regarding the effect of LFMV (frequency, ≤20 Hz) on OTM.
Recently, LFMV has gained interest in accelerating OTM by increasing alveolar bone turnover. The osteocytes in the bone tissue are thought to orchestrate “mechanotransduction” by reacting to different forms of mechanical loading through biologic signals. The role of osteocytes in bone remodeling and modeling has been well documented. It has been shown that osteocytes are the major source of sclerostin (product of SOST gene), and they antagonize the canonical Wnt signaling pathway, thus exhibiting an inhibitory effect on bone formation. Matsumoto et al demonstrated the role of osteocytes in resorption modeling during OTM (mechanotransduction) using osteocyte-ablated mice.
Our null hypothesis was that there would be no difference in the amount of tooth movement between different amounts of LFMV. We had 3 specific aims: (1) to determine the effect of LFMV on the rate of tooth movement; (2) to quantify bone modeling and remodeling in both the control and experimental groups using microfocus computed tomography (micro-CT) and immunostaining; and (3) to determine the effect of LFMV on the PDL.
Material and methods
The Institutional Animal Care Committee of the University of Connecticut Health Center approved this study, which conformed to the ARRIVE guidelines. Data were obtained from 64 male CD1 mice (Charles River Laboratories, Wilmington, Mass; body weight, 24-30 g). The 12-week-old mice were randomly divided into 2 groups: control and experimental. The control group was further subdivided into 4 groups; each control group had 5 mice: (1) group 1 (baseline), no spring and no mechanical vibration; (2) group 2, no orthodontic spring, but 5 Hz vibration was applied to the maxillary first molars; (3) group 3, no orthodontic spring, but 10 Hz vibration was applied to the maxillary first molars; and (4) group 4, no orthodontic spring, but 20 Hz vibration was applied to the maxillary first molars. The experimental group was also subdivided into 4 groups, each with 11 mice: (1) group 5, orthodontic spring only but no vibration; (2) group 6, orthodontic spring and 5 Hz vibration applied to the maxillary first molars; (3) group 7, orthodontic spring and 10 Hz vibration applied to the maxillary first molars; (4) group 8, orthodontic spring and 20 Hz vibration applied to the maxillary first molars. The animals were allowed at least a week of acclimatization at our Health Center to compensate for their different origins.
The animals were placed under general anesthesia with xylazine (13 mg/kg) and ketamine (87 mg/kg). A custom mouth-prop was fabricated from 0.036-in stainless steel wire and placed between the maxillary and mandibular incisors to hold the mouth open. A 0.004-in stainless steel ligature wire was passed beneath the contact between the maxillary first and second molars and threaded to the maxillary first molar ( Fig 1 , A ). A low force/deflection rate nickel-titanium coil spring (Ultimate Wireforms, Bristol, Conn) delivering 10 g of force was attached to the 0.004-in stainless steel ligature around the first molar, and the other end of the spring was attached to the incisors with 0.004-in stainless steel wire. The force/deflection rate for the spring was determined to calibrate the amount of force produced by activation of the nickel-titanium coil spring.
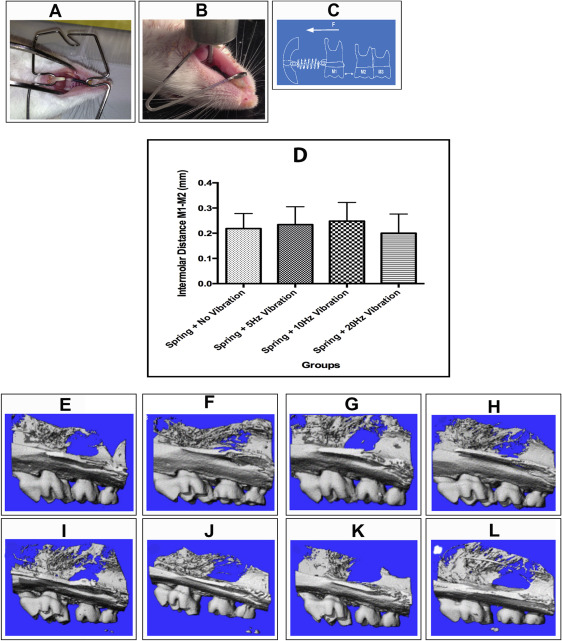
Additionally, grooves 0.5 mm from the gingival margin were prepared on the facial, mesial, and distal surfaces of the maxillary central incisors to prevent the ligatures from dislodging from the incisor because of their lingual curvature and eruption pattern. Self-etching primer (Transbond Plus; 3M Unitek, Monrovia, Calif) and light-cured adhesive resin cement (Transbond; 3M Unitek) were applied to the lingual surfaces of the maxillary first molars and incisors to secure the ligature wire. Moreover, to minimize the distal movement of the right incisor and to reinforce the anterior anchorage, the right and left incisors were joined together to act as a unit ( Fig 1 , A ). After appliance insertion, the mice were allowed to recover with an incandescent light for warmth; they were returned to their cages once full ambulation and self-cleansing returned. The appliances were checked every other day, and additional light-cured bonding material was added if necessary.
After anesthesia with ketamine (87 mg/kg) and xylazine (13 mg/kg), a custom mouth-prop fabricated from 0.017 × 0.025-in titanium-molybdenum alloy wire was placed between the maxillary and mandibular incisors to hold the mouth open. A feedback loop–controlled electromechanical actuator (model 3230; Bose Enduratec, Minnetonka, Minn) was used to apply unilateral LFMV to the occlusal surface of the maxillary right first molar along its long axis ( Fig 1 , B ). Loading protocols for each animal consisted of 15 minutes of LFMV at 1 cN of force with the electromechanical actuator at a frequency of 5, 10, or 20 Hz (cycles/second) depending on the mouse’s group. LFMV was applied to the maxillary right first molar at 3-day intervals (days 1, 4, 7, 10, and 13).
After the 14 days of the experimental period, the animals were killed by inhalation of carbon dioxide followed by cervical dislocation.
The maxilla was hemisected, and the attached soft tissues and muscles were removed. Subsequently, the hemisected maxilla was placed in 10% formalin for 5 days at 4°C. The samples were then decalcified in 14% ethylenediaminetetraacetic acid for 3 weeks and then processed for standard paraffin embedding. Serial sagittal sections (5 μm) were obtained from the mesial and distal roots of the maxillary right first molar.
All the animals in the different control (5 in each group) and experimental (11 in each group) groups were used to measure tooth movements. Three-dimensional image arrays of the hemisected right maxillae were collected using micro-CT. OTM was defined and measured as the distance between the maxillary first and second molars at the most mesial point of the second molar crown and the most distal point of the first molar crown. The distance at day 0 was 0 mm in all groups (ie, the convex crown surfaces were touching). The OTM measurements were done in the sagittal plane, locating the image plane that showed the most root structure. The original 2-dimensional image was then magnified 10 times for more precise line drawings, which were made at the closest proximity of the 2 convex molar crown surfaces. The OTM was measured in the 3 sagittal sections of each animal in all groups.
Micro-CT images were used for quantitative analyses of bone changes occurring in the region of the maxillary first molar. Changes in the alveolar bone were studied by analyzing the furcation area of the maxillary first molar. The region of interest for the alveolar bone analysis was defined vertically as the most occlusal point of the furcation to the apex of the maxillary first molar roots; transversely, it was defined as the space between the buccal and lingual cortical bone; sagittally, it included 50 sections (10 μm) beginning at the mesial root and continuing distally ( Fig 2 , A ). The parameters studied and measured using the established algorithms were bone volume fraction (BVF) and tissue density.
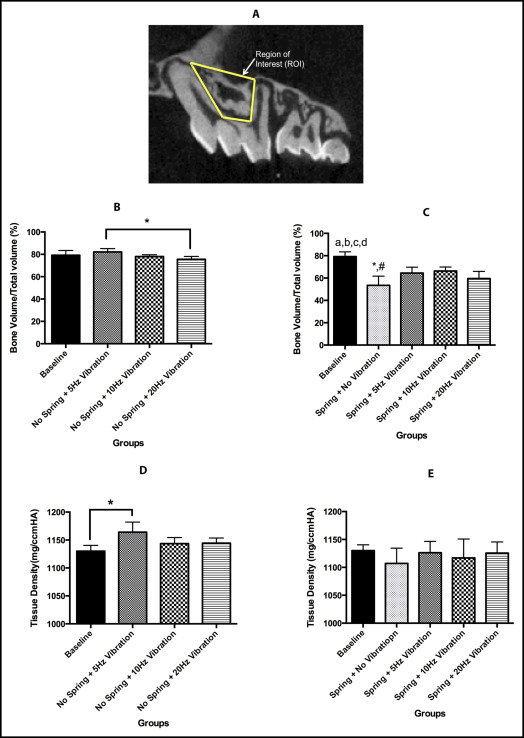
Histology, immunohistochemistry, tartrate-resistant acid phosphatase (TRAP), and picrosirius red staining histology were performed on each section. After blocking for 10 minutes at room temperature with 1x universal blocking reagent (HK085-5k; BioGenex, Fremont, Calif), deparaffinized histologic sections were incubated with goat polyclonal anti-SOST antibody (AF1589; R&D Systems, Minneapolis, Minn) overnight at +40°C at a concentration of 5 μg per milliliter. Subsequently, the sections were washed with phosphate-buffered saline and incubated with Alexa Fluor 594 donkey anti-goat IgG (A-11058; Life Technologies, Grand Island, NY) at a concentration of 1:300 for 1 hour at room temperature and mounted with a suspension of 50% glycerol in phosphate-buffered saline containing the nuclear stain (Hoechst H3570; Life Technologies) at a concentration of 1:5000.
TRAP staining was performed using a leukocyte acid phosphatase (TRAP) kit (386-1 KT; Sigma-Aldrich, St Louis, Mo) according to the manufacturer’s instructions. TRAP positive, multinucleated cells were counted on the alveolar bone surfaces on the mesial sides of the distobuccal roots. The area for quantification included a square with 1 side extending from the apex to the bifurcation, and the other side extending 200 μm from the PDL border inside the alveolar bone. The osteoclast numbers were counted in 6 sections from 4 mice in each group, and the values were then averaged for each animal to run a statistical test.
Deparaffinized sections were also stained with 1% picrosirius red for 1 hour, washed with acidified water (0.5% acetic acid water), and dehydrated with serial ethanol washes before mounting. The sections were examined with a fluorescent microscope (Carl Zeiss, Thornwood, NY).
Statistical analysis
Simple descriptive statistics were used to summarize the data. The outcomes examined included intermolar distance, BVF, and tissue density. Because of the sample size, nonparametric tests were used to examine the association between the outcome variables and the treatment groups (spring + no vibration, spring + 5 Hz, spring + 10 Hz, and spring + 20 Hz). The Kolmogorov-Smirnov test was used to examine the distribution of the outcome variables. Kruskal-Wallis tests were used to compare the outcomes across treatment groups. Pairwise comparisons between different groups were conducted with the Mann-Whitney U test. All statistical tests were 2 sided, and a P value of <0.05 was deemed to be statistically significant for the Kruskal-Wallis test. Because of the multiple pairwise comparisons used, to minimize type 1 errors, Bonferroni corrections were used.
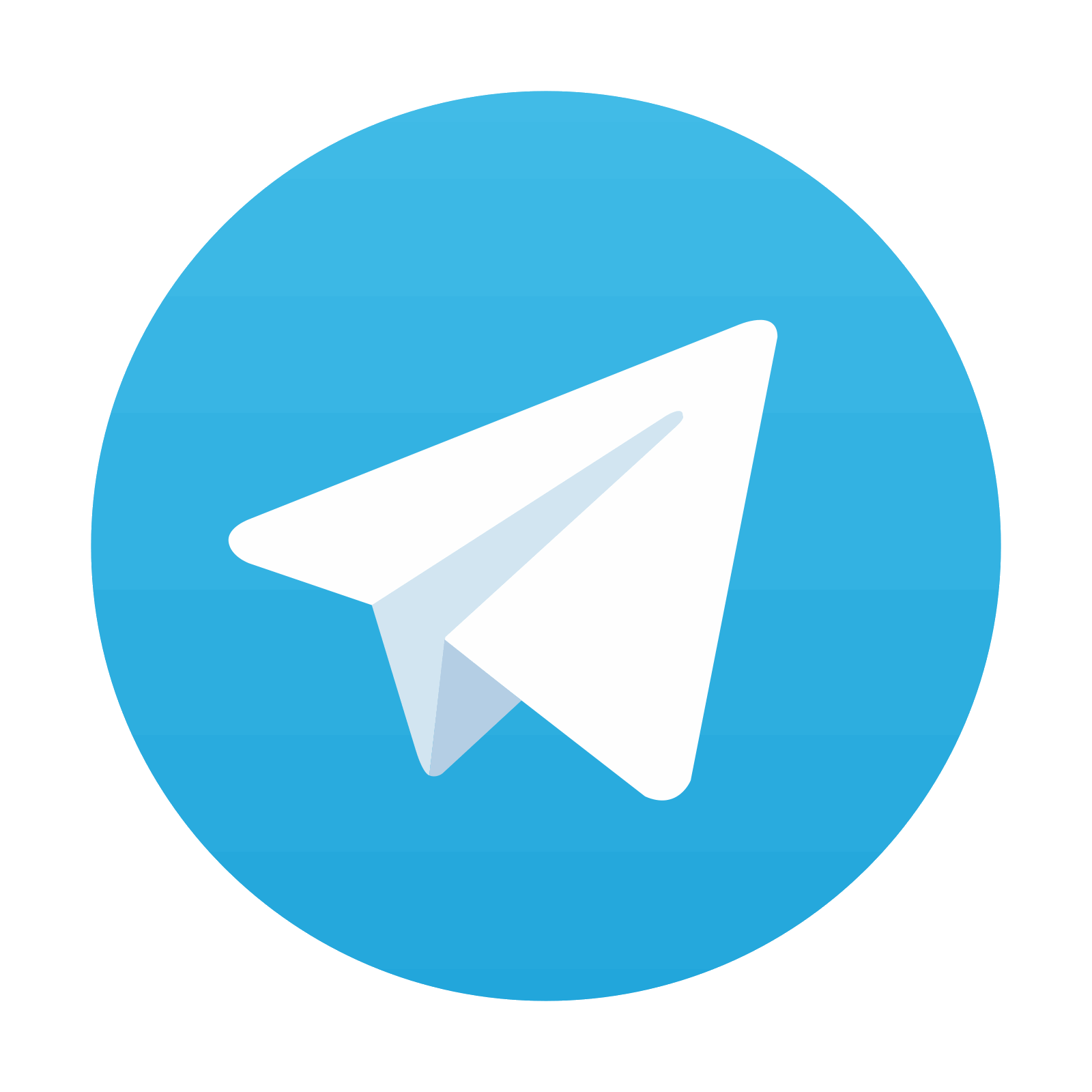
Stay updated, free dental videos. Join our Telegram channel

VIDEdental - Online dental courses
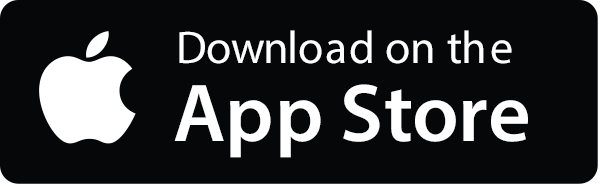
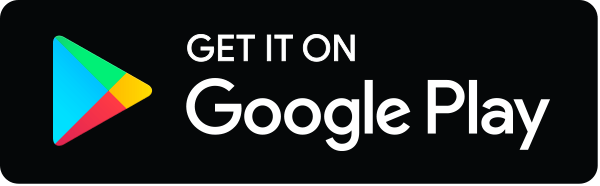