Highlights
- •
The possibility to use an ethanol-based cross-linker as a primer seems to be a promising option in terms of bond strength.
- •
N,N’-dicyclohexylcarbodiimide (DCC) pre-treatment efficiently preserves bond strength over time.
- •
Matrix-metalloproteinases activity seems to be influenced by the application mode of a universal adhesive.
Abstract
Objective
To evaluate the effects of N,N’-dicyclohexylcarbodiimide (DCC), an ethanol-based dentin cross-linker, on the immediate and long-term microtensile bond strength (μTBS) and nanoleakage expression of a universal adhesive employed in self-etch mode (SE) or etch-and-rinse mode (ER). The effect of DCC on the dentinal MMP activity was also investigated by means of in-situ zymography.
Methods
Eighty freshly extracted human molars were sectioned to expose mid-coronal dentin surfaces. The teeth were assigned to one of the following groups, according to the dentin surface priming/adhesive approach: (G1): DCC pre-treatment and Scotchbond Universal (SBU) in ER mode; (G2): SBU in ER mode; (G3): DCC pretreatment and SBU in SE mode; (G4): SBU in SE mode. μTBS test was performed immediately (T 0 ) or after 1-year aging (T 12 ) in artificial saliva. Ten additional teeth per group were prepared for nanoleakage evaluation (N = 5) and for in-situ zymography (N = 5).
Results
Three-factor analysis of variance revealed significant difference for the variables DCC pretreatment, application mode and aging (p < 0.05) for both microtensile bond strength testing and in-situ zymography. Nanoleakage analysis revealed reduced marginal infiltration of DCC experimental groups both at T 0 and T 12 .
Significance
The use of an ethanol-based primer containing DCC appears to be promising in preserving the stability of the adhesive interface of a universal adhesive, especially in the SE mode.
1
Introduction
Adhesion between resin composite restorations and dental substrate is achieved through the infiltration of resin monomers into the demineralized dentin collagen matrix after partial dissolution of the mineral inorganic phase [ ]. The need for a retentive cavity became less critical after the advent of adhesive dentistry, with dentin bonding systems provide reasonable immediate bond strength to the dental substrate [ , ]. Despite nearly six decades of refinement of adhesive materials and protocols, the interdiffusion area between dentin and the adhesive resin, known as the hybrid layer, still remains the weakest region of adhesively-based restorations [ ].
The potential reasons behind the degradation of resin-dentin bond over time had been examined by in vitro and in vivo studies. Hydrolytic or enzymatic breakdown of the polymerized resin compounds and endogenous protease-initiated degradation of the demineralized dentin collagen matrix have emerged as the most likely contributors of interfacial degradation [ ].
The dentin substrate contains collagen fibrils with bound non-collagenous proteins such as growth factors and endogenous proteases such as matrix-metalloproteinases (MMPs) and cysteine-cathepsin [ ]. Endogenous proteases play an important role during dentin maturation and become trapped and inactivated after mineralization of the collagen matrix [ ]. These enzymes are exposed and reactivated during demineralization of the mineralized dentin, progressively degrading the collagen fibrils that are not protected by adhesive resin within the hybrid layer, eventually resulting in the loss of retention of the adhesive restorations [ , ]. For this reason, much efforts have been devoted to increasing the resistance of the resin-sparse, water-rich collagen fibrils within the hybrid layer against MMPs, with the intention of increasing the longevity of adhesive restorations [ ].
Matrix metalloproteinases, particularly MMP-2 and MMP-9, are mostly responsible for the degradation of the dentinal organic matrix [ ]. For this reason, much efforts have been devoted to increasing the resistance of the resin-sparse, water-rich collagen fibrils within the hybrid layer against MMPs, with the intension of increasing the longevity of adhesive restorations [ , , , ].
Different MMP inhibitors such as quaternary ammonium methacrylates [ , ], and benzalkonium chloride [ ] have been used experimentally to increase the durability of the resin-dentin interface [ ]. Collagen cross-linkers have also been used to enhance the mechanical properties of the demineralized collagen network as well as bond durability in coronal [ , ] and radicular dentin [ ]. Because cross-linking of the collagen matrix is a naturally-occurring phenomenon in dentin, scientists have resorted to the use of chemical substances with cross-linking properties to render the dentin collagen matrix less susceptible to proteolytic attack [ , ].
Among the cross-linking reagents, 1-ethyl-3-(3-dimethylaminopropyl)carbodiimide (EDC), has demonstrated promising results in the preservation of the integrity of hybrid layers over time. This feature was attributed to the ability of EDC to cross-link peptides without introducing additional linkage groups [ , , ]. N,N’-dicyclohexylcarbodiimide (DCC) is a crosslinker belonging to the same family as EDC, but with a different solubility behavior. Whereas EDC is soluble in water, DCC is soluble in organic solvents such as ethanol or acetone [ ]. It is envisaged that the ethanol-based cross-linker agent may help counteract the deleterious effects of water during dentin bonding and preserve the hybrid layer.
Accordingly, the objective of the present in vitro study was to evaluate the ability of an ethanol solution of DCC to improve the bond strength of a universal adhesive employed either in self-etch or etch-and-rinse mode, and to stabilize the adhesive interfaces over time. The DDC was applied before adhesive procedures to cross-link dentinal collagen. The effect of DCC on dentin MMP activity was further investigated by in-situ zymography. The null hypotheses tested were that pre-conditioning of dentin with DCC prior to adhesive application 1) does not benefit the immediate bonding performance of a universal adhesive to dentin, 2) does not prevent interfacial degradation over time, and 3) does not inhibit endogenous dentin MMP activity.
2
Materials and methods
Freshly-extracted sound human third molars (N = 120) were obtained from anonymous individuals following their signed consent under the protocol ASL_BO N° 0013852, approved on 02/01/2019 by the Ethics Committee.
2.1
Microtensile bond strength test (μTBS)
Eighty teeth were selected to conduct μTBS testing. The occlusal surface of each tooth was cut transversely to the long axis to expose mid-coronal dentin using a low-speed diamond saw (Micromet, Remet, Bologna, Italy) with copious water cooling. A standardized smear layer was created with 600-grit silicon-carbide paper on each tooth surface. The polished teeth were randomly assigned to one of the following groups according to the dentin surface treatment and adhesive approach performed (N = 20; Table 1 ):
Material | Composition | ER mode | DCC + ER mode | SE mode | DCC + SE mode |
---|---|---|---|---|---|
Scotchbond Universal (SBU; 3M ESPE) | 1. Etchant: 32% phosphoric acid, water, synthetic amorphous silica, polyethylene glycol, aluminum oxide (Scotchbond Universal Etchant) 2. Adhesive: methacryloyloxydecyl dihydrogen phosphate (MDP) phosphate monomer, dimethacrylate resins, 2-hydroxyethyl methacrylate (HEMA), methacrylate-modified polyalkenoic acid copolymer, filler, ethanol, water, initiators, and silane | 1. Apply etchant for 15 s 2. Rinse for 10 s 3. Air dry 5 s 4. Apply the adhesive to the entire preparation with a microbrush and rub it in for 20 s 5. Direct a gentle stream of air over the liquid for 5 s until it no longer moves and the solvent is evaporated completely 6. Repeat steps 4 and 5 7. Light-cure for 20 s | 1. Apply 0.5M DCC ethanol-based primer and brush it for 1 min 2. Direct a gentle stream of air over the liquid for 5 s 3. Apply adhesive as for the ER mode | 1. Apply adhesive to the entire preparation with a microbrush and rub it in for 20 s 2. Direct a gentle stream of air over the liquid for 5 s until it no longer moves and the solvent is evaporated completely 3. Repeat steps 2 and 3 4. Light-cure for 20 s | 1. Apply first coat of adhesive 2. Apply 0.5M DCC ethanol-based primer and brush it for 1 min 3. Direct a gentle stream of air over the liquid for 5 s 3. Apply adhesive as for the SE mode |
Filtek Z250 (3M ESPE) | Triethyleneglycol dimetacrylate (TEGDMA) < 1–5%; Bisphenol-A-glycidylmethacrylate (Bis-GMA) < 1–5%; Bisphenol-A polyethylenglycol dietherdimethacrylate (Bis-EMA) 5–10%; Urethane dimethacrylate (UDMA) 5–10% Fillers: Zirconia/silica; 60 vol% inorganic fillers (particle size 0.01–3.5 μm) |
Group 1 (G1): DCC pre-treatment and Scotchbond Universal adhesive (SBU; 3M ESPE, St. Paul, MN, USA used in the etch-and-rinse mode (ER). The dentin surface was etched with 32% H 3 PO 4 (Scotchbond Universal Etchant, 3M ESPE) for 15 s, pre-treated with an ethanol solution of 0.5M DCC for 1 min, air-dried and bonded with SBU according to the manufacturer’s instructions ( Table 1 ).
Group 2 (G2): SBU in ER mode. No DCC was applied on dentin; SBU application was the same as G1.
Group 3 (G3): DCC pre-treatment and SBU in self-etch mode (SE). The dentin surface was pre-treated as in G1; instead of etching with 32% H 3 PO 4 , SBU was applied directly to the smear layer-covered dentin and agitated for 20 s. The adhesive was air-dried. Without light-curing, DCC was applied for 1 min. The second layer of universal adhesive was subsequently applied, air-dried and light-cured ( Table 1 ).
Group 4 (G4): SBU in SE mode. No DCC was applied on dentin. SBU application was the same as G3.
Each bonded specimen was light-cured for 20 s using a light-emitting diode curing light (Demi™ Plus, Kerr Corp., Brea, CA, USA) after solvent evaporation. Four 1-mm thick layers of a micro-hybrid resin composite (Filtek Z250; 3M ESPE) were incrementally placed over the bonded dentin and individually polymerized for 20 s each to obtain a 4-mm thick composite build up for μTBS testing. Each specimen was serially-sectioned to obtain approximately 1-mm thick sticks, each containing resin composite and dentin and with the adhesive interface in between, in accordance with the non-trimming technique of the μTBS test. The dimension of each stick (0.9 mm × 0.9 mm ± 0.01 mm) was recorded using a pair of digital calipers. The bonded area was calculated for subsequent conversion of microtensile strength values into units of stress (MPa). Sticks from each tooth were randomly assigned to two storage groups: 24 h (T 0 ) or 1 year (T 12 ) of storage in artificial saliva at 37 °C. The artificial saliva consisted of CaCl2 (0.7 mmoles/L), MgCl2 6H2O (0.2 mmoles/L), KH2PO4 (4.0 mmoles/L), KCl (30 mmoles/L), NaN3(0.3 mmoles/L) in HEPES buffer [ ].
Each stick was stressed to failure under tension using a simplified universal testing machine (Bisco, Inc., Schaumburg, IL, USA) at a crosshead speed of 1 mm/min. The number of prematurely-debonded sticks in each group was recorded, but those null values were not included in the statistical analysis. This is because all premature failures occurred during the cutting procedure and those failures did not exceed the 3% of the total number of tested specimens and were similarly distributed within the groups. A single observer evaluated the failure modes under a stereomicroscope (Stemi 2000-C; Carl Zeiss GmbH, Jena, Germany) at 30× magnification. Failure modes were classifieds adhesive failure (A), cohesive failure in dentin (CD), cohesive failure in composite (CC) or mixed failure (M).
Statistical analysis was performed using the tooth as the statistical unit. Bond strength data from each tooth were averaged to obtain the mean bond strength for that tooth. The acquired data were evaluated for compliance with the normality assumption using Shapiro-Wilk test, and the homoscedasticity assumption using the modified Levene test prior to the use of parametric analytical methods. A three-way analysis of variance (ANOVA) was performed to identify the effects of three variables, DCC pre-treatment (with/without), adhesive application mode (ER/SE) and aging (T 0 /T 12 ) and their interactions on bond strength. Post-hoc comparisons were conducted using Tukey test. Additionally, one-way ANOVA was conducted to evaluate differences within each variable. For all tests, statistical significance was pre-set at α = 0.05. Statistical analyses were performed using Stata 12.0 software for Mac (StataCorp, College Station, TX, USA).
2.2
Nanoleakage expression
Twenty teeth (N = 5) were used for examination of nanoleakage within the resin-dentin interface. Mid-coronal dentin were bonded in the same manner described for μTBS testing. Each specimen was cut vertically into 1-mm-thick slabs to expose the resin-dentin interface. After storage in artificial saliva at 37 °C for 24 h (T 0 ) or 12 months (T 12 ), the specimens were immersed in 50 wt.% ammoniacal AgNO 3 solution for 24 h in the dark, following the protocol described by Tay et al. [ ]. The specimens were then thoroughly rinsed in distilled water and immersed in a photo-developing solution for 8 h under a fluorescent light to reduce silver ions into metallic silver grains within voids along the bonded interfaces.
For light microscopy, the specimens were fixed, dehydrated, embedded in epoxy resin (LR White resin, MilliporeSigma, Burlington, MA, USA), fixed on glass slides using cyanoacrylate glue, flattened on a grinding device (LS2; Remet, Bologna, Italy) under water irrigation and polished with a graded series of silicon carbide abrasive papers of increasing fineness (180-, 600-, 1200-, 2400-, and 4000-grit). The presence of the silver tracer was examined along the bonded interface using light microscopy (E800; Nikon, Tokyo, Japan), at 20× magnification. Interfacial nanoleakage expression was scored by two trained investigators based on the percentage of adhesive surface showing AgNO 3 deposition, following the method of Saboia et al. [ ]. A scale 0–4 was used for evaluation: (0) no nanoleakage; (1) <25% surface with nanoleakage; (2) 25–50% surface with nanoleakage; (3) 50–75% surface with nanoleakage; and (4) >75% surface with nanoleakage. Intra-examiner reliability was evaluated using the Cohen’s kappa (κ) statistic. Statistical differences among nanoleakage scores were analysed with the chi-square statistic. Statistical significance was pre-set at α = 0.05.
2.3
In-situ zymography
Twenty freshly-extracted human third molars (N = 5) were used for in-situ zymography. One mm-thick slabs of middle/deep dentin were prepared. Each slab was further divided into four parts to test the 4 control land experimental groups on the same substrate ( Fig. 1 ). Silicon carbide paper (600-grit) was used to create a standardized smear layer on each dentin surface. One surface of each quarter of a slab was treated with the adhesive systems as described for μTBS testing.

The procedure was performed using the method previously reported by Mazzoni et al. [ , ]. After aging for the designated period (24 h or 1 year), each bonded slab was glued to a glass slide and polished to produce an approximately 40-μm thick section. To produce the substrate, 1.0 mg/mL of a stock solution containing self-quenched fluorescein-conjugated gelatin (E-12055; Molecular Probes, Eugene, OR, USA) was prepared by adding 1.0 mL deionized water to the vial containing the lyophilized gelatin. The substrate was stored at −20 °C until use. The gelatin stock solution was diluted 10 times with dilution buffer (NaCl 150 mm, CaCl 2 5 mm, Tris–HCl 50 mm, pH 8.0), followed by the addition of an anti-fading agent (Vectashield mounting medium with 4′,6-diamidino-2-phenylindole; Vector Laboratories, Burlingame, CA, USA). Then, 50 μL of the fluorescent gelatin mixture was placed on top of each polished dentin section and protected with a cover slip. The glass slide assemblies were light-protected and incubated in a humidified chamber at 37 °C for 48 h.
Detection of endogenous gelatinolytic enzyme activity within the hybrid layer was based on hydrolysis of the quenched fluorescein-conjugated gelatin substrate. The process was evaluated by examining the glass slides with a multi-photon confocal laser scanning microscope (LSM 5 Pa; Carl Zeiss), using an excitation wavelength of 495 nm and an emission wavelength of 515 nm. Samples were imaged using a HCX PL APO 40×/1.25 NA oil immersion objective. Series of x-y-z images (0.145*0.145*1 μm3 voxel size) were collected. Laser power and detector gain were set at the beginning of the experiment and kept the same for all specimens in order to have the possibility to compare different groups. Sixteen to 20 optical sections were acquired for each specimen. The stacked images were analyzed, quantified, and processed with ZEN 2009 software (Carl Zeiss). The fluorescence intensity emitted by the hydrolyzed fluorescein-conjugated gelatin was isolated and quantified using Image J (ImageJ; National Institute of Health, Bethesda, MD, USA). The amount of gelatinolytic activity was expressed as a percentage of the green fluorescence within the hybrid layer.
Negative control sections were similarly incubated, with the exception that 250 mL ethylenediaminetetraacetic acid (EDTA) or 2 mM 1,10-phenanthroline was dissolved in the mixture of quenched fluorescein-conjugated gelatin. The EDTA- and 1,10-phenanthroline-containing gelatin were used as negative controls. In addition, standard non-fluorescent gelatin was used as the third negative control.
Because the in-situ zymography data complied with normality and homoscedasticity assumptions after non-linear transformation, a three-way ANOVA was used to identify the effects of the three variables, DCC pre-treatment, adhesive application mode and aging, on the density of fluorescence signals. Additional one-way ANOVA was conducted to evaluate differences within each variable. Statistical significance was pre-set at α = 0.05.
3
Results
3.1
Microtensile bond strength
Microtensile bonds strengths of the four groups tested at T 0 and T 12 are summarized in Table 2 . Three-factor ANOVA revealed significant difference for the variables DCC pre-treatment, adhesive application mode and aging (p < 0.05), as well as for the interaction between DCC pre-treatment and adhesive application mode (p < 0.05). Post-hoc comparisons showed that the use of a 0.5 M DCC-containing ethanol solution before adhesive application improved bond strength of SBU (G1 and G3) vs control groups (G2 and G4) (p < 0.05), irrespective of the adhesive application mode and aging. Additionally, SBU generated higher bond strength when employed in the ER mode vs the SE mode (p < 0.05). Aging significantly reduced μTBS among all the adhesive application mode/dentin pre-treatment combinations, except for G3 (p < 0.05). One-way ANOVA indicated that at T 0 , DCC pre-treatment significantly improved μTBS in both experimental groups compared to the control groups (G1 46.0 ± 15.3; G2 37.1 ± 12.5; G3 39.4 ± 11.1; G4 26.3 ± 11.4). After aging, DCC pre-treatment showed a preservation of the bond strength when SBU was applied in the SE mode (T 0 = 39.4 ± 11.1 and T 12 = 35.3 ± 13.9) thus, shoving a final MPa value after aging comparable to that of the SBU ER groups. For all groups at T 0 or T 12 the predominant failure modes were the adhesive failure and the mixed failure. At baseline, for all groups, except for G2 the adhesive failure were around 70% of the failure. After aging, the number of mixed failure increased between 40% and 55% in the different groups ( Table 3 ).
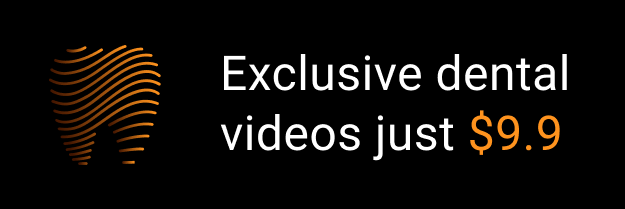