Highlights
- •
Treatment to obtain superhydrophile surfaces increases surface roughness and area.
- •
Insertion into low- density artificial bone tends to equalize surface roughness parameters.
- •
Surface treatment affects the amount of Ti particles released during implant insertion.
- •
Benchtop micro X-Ray Fluorescence (μ-XRF) spectrometers effectively detect Ti along the bone bed.
Abstract
Objective
To evaluate the influence of hydrophilicity on the surface integrity of implants after insertion in low-density artificial bone and to determine the distribution of titanium (Ti) particles along the bone bed.
Methods
Forty-eight dental implants with different designs (Titamax Ex, Facility, Alvim, and Drive) and surface treatments (Neoporos® and Aqua™) were inserted into artificial bone blocks with density compatible with bone type III-IV. Hydrophobic Neoporos® surfaces were obtained by sandblasting and acid etching while hydrophilic Aqua™ surfaces were obtained by sandblasting, acid etching, and storage in an isotonic 0.9% NaCl solution. The surface integrity was evaluated by Scanning Electron Microscope (SEM) and the surface roughness parameters (Sa, Sp, Ssk, Sdr, Spk, Sk, and Svk) and surface area were measured with Laser Scanning Confocal Microscopy before and after installation. Bone beds were inspected with Digital Microscopy and micro X-Ray Fluorescence (μ-XRF) to analyze the metallic element distribution along the bone bed.
Results
Acqua™ implants had higher initial Sa and a pronounced reduction of Sa and Sp during insertion, compared to NeoPoros® implants. After insertion, Sa and Sp of Acqua™ and NeoPoros® implants equalized, differing only between designs of Acqua™ implants. Surface damage was observed after insertion, mainly in the apical region. Facility implants that are made of TiG5 released fewer debris particles, while the highest Ti intensity was detected in the cervical region of the Titamax Ex Acqua™ and Drive Acqua™ implants.
Significance
Physicochemical modifications to achieve surface hydrophilicity created a rougher surface that was more susceptible to surface alterations, resulting in more Ti particle release into the bone bed during surgical insertion. The higher Ti intensities detected in the cervical region of bone beds may be related to peri-implantitis and marginal bone resorption.
1
Introduction
Oral rehabilitation with titanium (Ti) implants routinely achieves survival rates of ≥94% over 10 years of follow-up [ ]. The biocompatibility and corrosion resistance due to the stable superficial oxide layer [ ] combined with the surface microtopography [ ] and implant geometry [ ] are responsible for the successful osseointegration [ ] and long-term mechanical stability of Ti implants. At the same time, studies showing high prevalence rates of peri-implantitis [ ], progressive marginal bone loss [ ], and high risk of implant failure in low-density bone [ , ] highlight that field of implant dentistry still faces challenges that require further innovation.
New implant designs, surface treatment procedures [ ], and surgical milling protocols [ ] primarily focus on maximizing and accelerating osseointegration to reduce the potential for implant failure. For this reason, modification of topographical features and optimization of surface roughness has been the focus of research on implant surfaces over the last decades [ ]. Surface roughness affects initial events of osseointegration such as cell adhesion, proliferation, and differentiation, directly influencing biomaterial-bone interaction [ , , ]. Implants with a moderately rough surface (Sa = 1–2 μm) are more attractive for new bone formation, presenting a higher bone-implant contact rate (BIC) and removal torque than those with smooth (Sa < 0.5 μm) or rough (Sa > 2 μm) surfaces [ , ].
Currently, much research focuses on the interaction between topographic features and wetting properties through physicochemical modifications [ ]. These modifications jointly influence the topographic, mechanical, and surface chemical properties, resulting in varying surface roughness, increasing surface energy, reducing surface tensions [ ], changing surface charge and chemical composition, which induces surface wettability and bioactivity [ , ] and may also increase resistance to the shear forces generated during implant insertion [ ]. Wettability is quantified by the liquid–solid contact angle (Ɵ), and water Ɵ is classified as follows: superhydrophilic (Ɵ very close to 0°), hydrophilic (Ɵ < 90°), hydrophobic (Ɵ > 90°), and superhydrophobic (Ɵ > 150°) [ ].
The synergism of micro and nano-roughness with surface wettability influences the molecular and cellular interactions of the implant surface with the bioliquids (e.g., blood), optimizing and accelerating the initial bone healing period [ , , ]. Studies have shown that hydrophilic implants show superior clinical results than hydrophobic implants in the early bone healing period with higher BIC [ ], bone area fraction occupied [ , ] and primary stability [ ], which indicates their suitability for critical situations, such as in cases with low density bone and immediate loading [ , ].
The SLActive® surface (Straumann®, Basel, Switzerland) is the most widely investigated superhydrophilic surface [ ]. More recently, the Acqua™ surface (Neodent®, Curitiba, PR, Brazil) has been made available on the market [ , ]. Both surfaces are created by abrasive sandblasting, combined with acid-etching and rinsing for surface neutralization in a protective nitrogen environment [ , ]. The obtained surface has a high surface energy, a positive surface charge, and a lower Ɵ, and is stored in an isotonic 0.9% NaCl solution [ , ] to preserve the surface hydroxylation and to avoid contamination with organic components and carbonates until installation [ , ].
From a tribological point of view, wettability is associated with the application of lubricant fluids between the contact surfaces and relative motion. The primary objective of lubrication is to prevent contacts between asperities on both surfaces, reducing the coefficient of friction and surface wear [ ]. Rupp et al. suggested that bioliquids dynamically wet biomaterials during relative movements, such as when implants are inserted into the blood-filled surgical wound [ ]. Blood is a viscous fluid constituted of plasma, an aqueous solution containing salts, gases, proteins, amino acids, and glycoproteins. Proteins and erythrocyte volume determine blood viscosity, which influences the formation of a lubricating film [ ]. In addition, the apparent viscosity of blood depends on the shear rate [ ]. Shear stress is generated from implant friction against bone during insertion, creating a dynamic stress concentration along the bone-implant interface, causing bone rupture [ ], surface damage to the implants and release of wear particles in the peri-implant region [ ]. The surface area at the bone-implant interface affects the insertion torque and directly relates to roughness and implant geometry [ ].
During the insertion of implants, Ti particles are assumed to be released [ , ] . In vitro studies revealed damage to implant surfaces and the presence of Ti particles in the bone bed after implant insertion [ ]. Surfaces with higher average roughness and predominance of peaks showed a greater reduction of roughness parameters and an increased Ti particle release [ , ]. However, there are currently few studies that investigated the impact of hydrophilicity on the implant surface integrity after insertion [ ].
Ti particles are commonly detected around peri-implant tissues with the highest Ti concentration at the bone-implant interface [ ]. At present, there are no studies that map Ti intensity along the bone bed using a benchtop micro X-Ray Fluorescence (μ-XRF) spectrometer. Ti debris can generate an exacerbated inflammatory process with increased expression of pro-inflammatory cytokines related to the osteolytic process [ ]. Recent studies suggested that Ti particles at the bone-implant interface may influence the pathogenesis of peri-implantitis diseases and marginal bone resorption [ ]. The evaluation of the deleterious effects of surface wear and Ti particles release by hydrophilic implants is difficult to assess since studies that quantify the surface integrity of hydrophilic implants [ ] and the spatial distribution of Ti intensity around the implant by Synchrotron Radiation X-Ray Fluorescence (SRXRF) spectrometer are still scarce [ , , ], in part because access to SRXRF is limited. Therefore, the aim of this study was to (1) analyze the effect of surface hydrophilicity of implants with different geometries on surface integrity after insertion, (2) to evaluate the Ti intensity distribution along the bone bed, and (3) to establish the benchtop μ-XRF system as an accessible methodology for spatial analysis of metallic particles.
2
Materials and methods
2.1
Experimental design
This in vitro study evaluated the influence of hydrophilicity on surface changes after implant installation in artificial bone blocks (Sawbones, Pacific Research Laboratories Inc., Washington, DC, USA) with density compatible with bone type III and IV (combination of block grade 20 (0.32 g/cm 3 ) and sheets grade 40 (0.64 g/cm³). Forty-eight commercial implants (Neodent®, Curitiba, PR, Brazil) with 4 different designs and 2 surface treatments were investigated (6 implants per group): 1 – Titamax Ex: cylindrical body with V-shaped double thread, 2 – Facility: cylindrical body with spiral-shaped double thread, 3 – Alvim: tapered body with buttress-shaped double thread, and 4 – Drive: tapered body with double and progressive thread with buttress-shape in the cervical region transitioning to V-shaped threads in the apical region and counterclockwise cutting chambers ( Fig. 1 (a–d)).

The hydrophobic NeoPoros® surface was the control group and the superhydrophilic Acqua™ surface was the experimental group. Both surfaces are produced sandblasting with abrasive particles followed by acid etching. The physically and chemically activated Acqua™ surface is characterized by an electro-positive Ti oxide layer and stored in an isotonic 0.9% NaCl solution. All implant types were made of pure grade IV Ti and had Morse taper connections, diameters of 3.5 mm, and lengths of 13 mm, except the Facility implants that are composed of Ti alloy (Ti-6AL-4V), and have diameterof 2.9 mm and lengthof 12 mm. The surface roughness was the primary outcome and was measured by 3D Laser Scanning Confocal Microscope (LSCM) using the X20 objective to determine the following parameters: Sa, Sp, Ssk, Sdr, Spk, Sk, and Svk. The surface integrity was subsequently evaluated by Scanning Electron Microscope (SEM) in the cervical, central, and apical regions of the implants. In addition, the three-dimensional (3D) surface topography of the thread top in the cervical region of the implants was observed by LSCM with the X50 objective. The surface area of the implants was also analyzed using LSCM using the X10 objective. All analyses were performed before and after the insertion of the implants in the artificial bone. After implant installation, the artificial bone blocks were separated and inspected with a Digital Microscope (DM) using the X35 objective and with a μ-XRF spectrometer to investigate the distribution of metallic particles along the bone bed.
2.2
Implant installation
The implants were inserted into blocks of artificial bone (rigid polyurethane foam blocks – Sawbones, Pacific Research Laboratories Inc., Washington, DC, USA) with densities similar to maxillary bone. Rigid polyurethane foam (RPF) has a uniform density, consistent mechanical properties comparable with human cortical and cancellous bone, and a closed-cell structure similar to cancellous bone [ , ]. The low density artificial bone used in this study is a combination of 20 Process Classification Framework (PCF) RPF blocks with a density of 0.32 g/cm 3 that simulate the spongy bone and 1.5 mm of thick solid 40 PCF RPF with a density of 0.64 g/cm 3 that simulate the cortical bone [ , , ]. The custom block (170 × 120 × 46 mm) was produced in accordance with ASTM F1839-08 [ ]. Forty-eight blocks with dimensions approximately of 15 × 15 × 20 mm were cut transversely in two halves with a diamond blade (IsoMet Diamond Wafering Blades, Buehler, IL, USA)( Fig. 2 a) [ , ] and randomly divided in eight groups.

The drilling and implant installation were performed by a calibrated operator. The two halves of each block were joined with a bench vice for drilling ( Fig. 2 b) [ ]. The drilling sequence was performed as recommended by the manufacturer, using a surgical motor (Implantmed Plus SI-1023 – W&H Dentalwerk Bürmoos GmbH, Bürmoos, Austria) with a 20:1 contra-angle transmission. The drilling speed was 800 rotations per minute (rpm) and the speed of insertion was 30 rpm. The maximum torque during insertion was fixed at 60 N.cm. The implants were placed 2 mm infra-osseous, as indicated by Morse taper connection ( Fig. 2 c). The bench vice was removed after implant installation and the blocks were opened, allowing implant removal without counter torque from the bone bed ( Fig. 2 d). The two halves of each block were stored individually for the bone bed analysis.
The opening of the blocks allowed to preserve of the implant surface during their removal from the bone bed. The implants were cleaned with distilled water (30 min) and acetone (10 min) in an ultrasonic bath (UltraCleaner 1400, Unique, Indaiatuba, SP, Brazil) to remove any polyurethane debris [ ]. Implant surfaces were analyzed at the same sites before and after insertion [ ], using scratch marks on the implant platform and on a screwdriver ( Fig. 3 a). Implants with Acqua™ surface were dried with compressed nitrogen gas before analysis.

2.3
Surface analysis
A SEM (JEOL JSM-IT300LV, Akishima, Tokyo, Japan) was used to compare the surface integrity before and after insertion. SEM images of the thread tops ( Fig. 3 b) were made at X1500 magnification and an acceleration voltage of 15 kV in cervical, central and apical regions of the implant ( Fig. 3 c). The LSCM (3D VK-X200 LSCM, Keyence Corporation, Osaka, Japan) from the National Nanotechnology Laboratory (LNNano, Campinas, SP, Brazil) was used to characterize the surface topography [ ]. A LSCM with laser beam light source (violet laser light; 408 nm wavelength) generated high-resolution 3D images (800k pixels) using focused Z-axis scanning. 3D images of each implant type before and after insertion were taken with objective X10 (0.30 μm diameter), depth resolution (Z pitch) of 2.0 μm at a light intensity (ND filter) of 100%. The laser beam perpendicular to the surface is incapable of scanning the lowest part of the implant. Each half the implant diameter was longitudinally scanned in order to create the 3D image on both implant sides. Surface area of the implants was measured with a VK-X100 K/X200 K Analyzer (Keyence Corporation, Osaka, Japan) ( Fig. 3 d). The X50 objective (0.80 μm diameter), a Z pitch of 0.010 μm and a 10% ND filter, was used in the comparative topographic analysis of the thread tops in the cervical region ( Fig. 3 (b, c)). However, topographic investigation in other regions was not possible due to the curvature of the implant designs, as this would compromise the focus and quality of the 3D scan. The X20 objective (0.46 μm diameter), a Z pitch of 0.1 μm, and a ND filter of 30% was used during the surface roughness analysis. These measurements were performed in the top, flank and valley [ ] of the threads in the cervical, central, and apical regions of the implant [ ], totaling 27 measurements per implant ( Fig. 3 (b, c)). The large quantity of data obtained increases the representativity of the data for the entire screw thread [ ].
The surface topography consists of form, waviness, and roughness [ ], so a 50 × 50 μm Gaussian filter (ISO 11562:1996[ , ] was applied to remove errors of form and waviness [ ]. Data from the measuring area (40 μm × 80 μm) [ ] were processed with the ISO-25,178 Surface Texture Measurement Module of the VK-X100 K/X200 K Analyzer software used to calculate the roughness parameters. The selected 3D roughness parameters (ISO 25178-2:2012) [ ] were:
- •
Amplitude parameters: S a = arithmetical mean height; S sk = skewness (degree of symmetry of the surface heights about the mean plane); S p = maximum peak height;
- •
Hybrid parameter: S dr = interfacial area ratio;
- •
Functional parameters: S pk = peak height above the core roughness (reduced peak height); S k = core roughness height of the surface with the predominant peaks and valleys removed (core roughness depth); S vk = valley depth below the core roughness (reduced valley depth).
2.4
Bone analysis
The bone beds were inspected with a DM (3D Digital Microscope KH-8700, Hirox, Hackensack, NJ, USA) using X35 objective to check for debris particles resulting from surface damage during insertion. The metallic element distribution along the bone beds was determined with a benchtop μ-XRF spectrometer (Orbis Micro-XRF Analyzers, EDAX Inc, Mahwah, NJ, USA) in the Laboratory of Nuclear Instrumentation, Center of Nuclear Energy in Agriculture (CENA), University of São Paulo (USP), Brazil. The analyses were carried out using a Rh X-Ray tube at 30 keV and 500 μA, a collimator of 1 mm, a dwell time of 30 s and the X-Ray sample spectrum was recorded by an X-Ray silicon drift detector. Subsequently, a line scan analysis was used to characterize the metallic element distribution along the cervical, central, and apical regions of bone beds (cf. Supplementary material). Sixteen points were investigated along the length of the bone bed (approximately 12–13 mm). A limit of intensity (LOI) was determined by Eq. (1) to distinguish the Ti, aluminum (Al), and Iron (Fe) XRF signal from the background noise, values below the LOI were eliminated. Also using Orbis Vision V2.1 software (EDAX Inc, Mahwah, New Jersey, USA), the Ti, Al and Fe presence was confirmed by spectral verification.
Where: LOI (cps) = limit of intensity; BG (cps) = average background; t (s) = dwell time.
2.5
Statistical analysis
The roughness parameters were submitted to a two-way ANOVA, Student’s t-test; and post hoc Tukey tests (SAS version 9.0, SAS Institute, Cary, NC, USA). The significance level was set at 5%.
3
Results
3.1
Surface topography characterization
The implant insertion torque (IT, Ncm) means ± standard deviation were 39.40 (±3.34), 20.00 (±2.54), 45.20 (±3.91) and 45.50 (±6.43) for Titamax Ex, Facility, Alvim, and Drive implants with NeoPoros® surface, respectively; and 42.00 (±2.11), 14.00 (±3.20), 49.70 (±5.21) and 51.70 (±5.17) for Titamax Ex, Facility, Alvim and Drive implants with Acqua™ surface, respectively.
The mean values and associated standard deviations are shown in Table 1 , along with the relative reduction in the roughness parameters Sa and Sp. Surface treatment significantly influenced the Sa values of implants before insertion. Acqua™ implants had higher Sa values than NeoPoros® implants (p < 0.05). The macrogeometry significantly affected the Sa values of the Neoporos® implants and the Sp values for Acqua™ implants. There was a significant reduction in Sa and Sp values after bone insertion for all implants (p < 0.05). After insertion, Sa and Sp values of both surface types were similar (p > 0.05), and differences were only present between implants designs with the Acqua™ surface (p < 0.05). The Sa reduction percentage (Δ%) was considerably higher in Acqua™ surface implants, especially for Drive (Δ 45.97%), Titamax EX (Δ 28.71%), and Alvim (Δ 26.42%) implants. These same implants with NeoPoros® surfaces had a higher Sa value before insertion and a higher Δ% after insertion when compared with Facility NeoPoros® (Δ 4.44%). Similarly, the Sp Δ% was more pronounced in Acqua™ surface implants, especially in Drive (Δ 44.77%) and Titamax Ex (Δ 32.84%) implants.
Amplitude parameter | Implant | Before | After | Percentage of reduction | |||||||
---|---|---|---|---|---|---|---|---|---|---|---|
Neoporos® | Acqua™ | Neoporos® | Acqua™ | Neoporos® | Acqua™ | ||||||
Sa (μm) | Titamax Ex | 0.87 ± 0.58 | Bab | 0.92 ± 0.51 | Aa | 0.69 ± 0.57 | Aa* | 0.60 ± 0.29 | Abc* | 15.45% | 28.71% |
Facility | 0.77 ± 0.50 | Bb | 1.05 ± 0.61 | Aa | 0.69 ± 0.64 | Aa* | 0.80 ± 0.41 | Aa* | 4.44% | 15.55% | |
Alvim | 0.97 ± 0.35 | Ba | 1.03 ± 0.47 | Aa | 0.75 ± 0.29 | Aa* | 0.66 ± 0.22 | Ab* | 15.86% | 26.42% | |
Drive | 0.92 ± 0.41 | Bab | 1.15 ± 0.46 | Aa | 0.63 ± 0.20 | Aa* | 0.53 ± 0.20 | Ac* | 22.34% | 45.97% | |
Sp (μm) | Titamax Ex | 2.80 ± 2.49 | Aa | 2.59 ± 1.67 | Ab | 2.01 ± 1.73 | Aa* | 1.55 ± 0.94 | Ab* | 12.33% | 32.84% |
Facility | 2.40 ± 1.72 | Aa | 3.50 ± 2.76 | Aa | 1.86 ± 1.32 | Aa* | 2.12 ± 1.26 | Aa* | 8.30% | 27.99% | |
Alvim | 2.54 ± 1.14 | Aa | 2.89 ± 1.92 | Aa | 2.35 ± 1.34 | Aa* | 1.97 ± 0.99 | Aa* | 2.17% | 16.30% | |
Drive | 2.46 ± 1.42 | Aa | 3.28 ± 1.63 | Ab | 1.85 ± 0.74 | Aa* | 1.49 ± 0.75 | Ab* | 11.11% | 44.77% |
The Ssk parameter did not change after implant installation (p > 0.05) ( Fig. 4 (a–d)). The only significant difference in Ssk values between NeoPoros® and Acqua™ surfaces was observed in the cervical region of the Titamax Ex implant before insertion (p < 0.05) ( Fig. 4 a).

The Sdr parameter changed significantly after insertion for all implants (p < 0.05) ( Fig. 5 (a–d)). The Acqua™ surface had significantly higher Sdr values before insertion and showed significantly lower values than the Neoporos® surface in some regions after insertion (p < 0.05). The Facility implants with Acqua™ surfaces showed lower Sdr values than those with Neoporos® surfaces in all three regions after insertion (p < 0.05) ( Fig. 5 b).

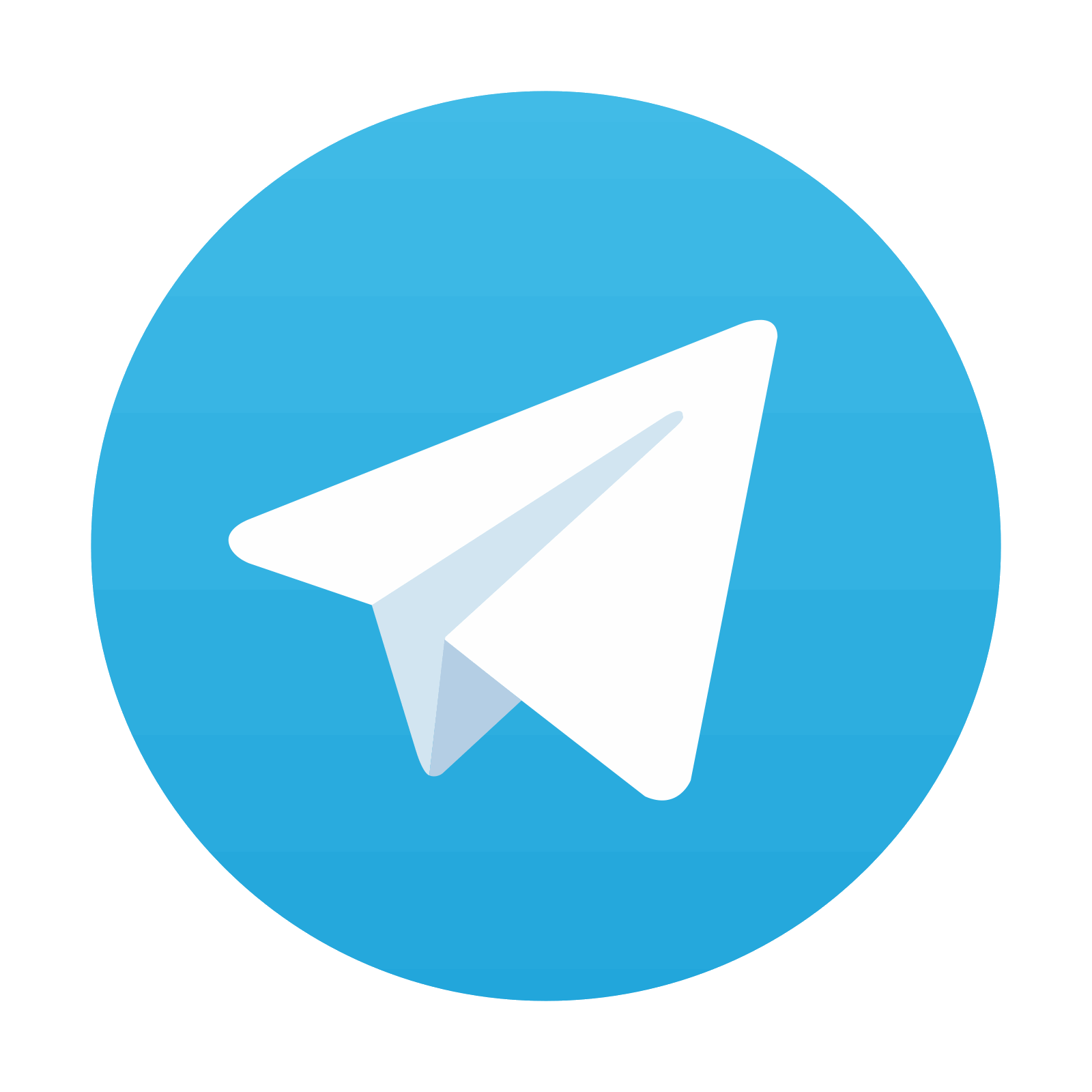
Stay updated, free dental videos. Join our Telegram channel

VIDEdental - Online dental courses
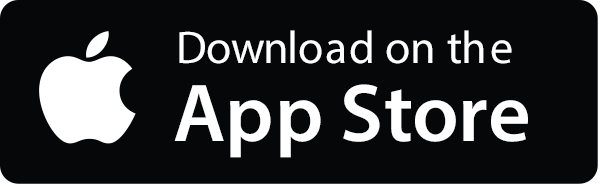
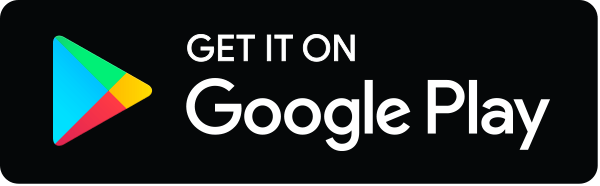
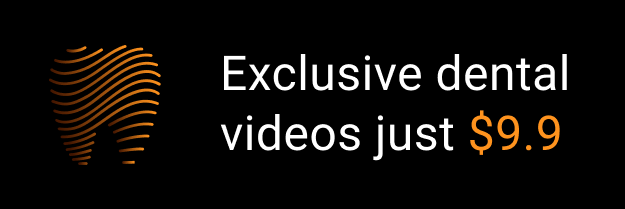