Abstract
Objective
Knowledge of the structural organization and mechanical properties of dentin has expanded considerably during the past two decades, especially on a nanometer scale. In this paper, we review the recent literature on the nanostructural and nanomechanical properties of dentin, with special emphasis in its hierarchical organization.
Methods
We give particular attention to the recent literature concerning the structural and mechanical influence of collagen intrafibrillar and extrafibrillar mineral in healthy and remineralized tissues. The multilevel hierarchical structure of collagen, and the participation of non-collagenous proteins and proteoglycans in healthy and diseased dentin are also discussed. Furthermore, we provide a forward-looking perspective of emerging topics in biomaterials sciences, such as bioinspired materials design and fabrication, 3D bioprinting and microfabrication, and briefly discuss recent developments on the emerging field of organs-on-a-chip.
Results
The existing literature suggests that both the inorganic and organic nanostructural components of the dentin matrix play a critical role in various mechanisms that influence tissue properties.
Significance
An in-depth understanding of such nanostructural and nanomechanical mechanisms can have a direct impact in our ability to evaluate and predict the efficacy of dental materials. This knowledge will pave the way for the development of improved dental materials and treatment strategies.
Conclusions
Development of future dental materials should take into consideration the intricate hierarchical organization of dentin, and pay particular attention to their complex interaction with the dentin matrix on a nanometer scale.
1
Introduction
The field of restorative dentistry has evolved considerably in the past two decades. New restorative methods have been proposed, the concept of regenerative dentistry has been brought forward, and existing dental materials have increasingly become more biocompatible , bioactive and biomimetic . Key to such developments has been a profoundly more in-depth understanding of the tissues that compose the tooth at smaller length-scales . This has been made possible by the fast development of characterization tools and techniques that can probe tissue structures at ever-finer scales.
Dentin is the largest structure in the tooth, and is composed of hydroxyapatite mineral crystallites, collagen fibrils (mostly type I) and noncollagenous macromolecules . A great number of current restorative procedures use dentin as a substrate, therefore, the success of restorative dental materials invariably depends on a thorough understanding of the structural and mechanical property relationships that characterize the dentin matrix. Moreover, dentin, together with dental enamel, represents a unique biomaterial in nature, in that it retains outstanding durability despite the life-long cyclic loading imposed onto the tooth, and the absence of cell-regulated mechanisms of remodeling or repair that are common in other tissues. Therefore, dentin offers a classic example of how nature designs stiff and tough natural biomaterials with outstanding longevity, simply by combining soft (proteins) and rigid (mineral) building blocks at precisely organized hierarchical levels . Thus, if from a reverse engineering standpoint the ultimate goal of restorative dental materials is to closely approximate the longevity and properties of the tooth, knowledge of the mechanisms endowing dentin with its outstanding properties is imperative.
In this review we cover recent findings regarding the mechanical and structural relationships of dentin on the nanoscale. We adopt a hierarchical approach to explore aspects that relate to both tissue nano-structure and nano-mechanics, and the relative participation of the inorganic and organic constituents that make up the tissue matrix. Regarding the former, we discus the specific of the intrafibrillar mineral to the mechanical properties of dentin. On the latter, we cover the specific structural and mechanical contributions of collagen and, more in depth, non-collagenous nanoscale components, as determined by various analytical methods. Additionally, we offer specific insights into the challenges and limitations of existing dental materials and treatments (i.e. remineralization and bonding), especially in relation to the complexity of dentin proteins on the nanometer and molecular length scales. Finally, we provide a forward-looking overview of the gradual transition of dentistry into the emerging fields of bioinspired materials engineering and regeneration. We discuss how these emerging topics will influence the development of novel dental materials with properties that more closely approximate those of the natural structures in the tooth, and hence will provide impetus for the shift of clinical dentistry from reparative-based treatment methods to more biologically-assisted regenerative approaches.
2
Dentin inorganic content—extrafibrillar and intrafibrillar mineral
During development, differentiated odontoblasts secrete the dentin matrix in a well orchestrated sequence that is characterized by the release of collagen fibrils with high concentrations of carboxylated non-collagenous proteins and proteoglycans . These matrix constituents, respectively, regulate the process of mineral deposition and fibrillogenesis during dentin formation. The process of collagen biomineralization has been a topic of much debate in the recent literature. Although it has long been acknowledged that collagen mineralization occurs due to mineral release from vesicles in the extracellular matrix (which have also been called calcospherites early on), it has recently been demonstrated that prior to secretion, the mineral accumulates intracellularly in the mitochondria , and travels to the extracellular space where it accumulates calcium while it remains in the form of amourphous calcium phosphate until full release onto the collagenous network .
Extensive work has shown that acidic non-collagenous proteins of the SIBLING family assist in stabilizing the calcium phosphate in the amorphous phase, aiding the penetration of the amorphous mineral in the gap zones and intermolecular spaces of collagen fibrils via capillary forces and osmotic-regulated mechanisms . Subsequent phase transformations allow the amorphous mineral to adopt a more crystalline and stable hydroxyapatite morphology , and form what is known as the intrafibrillar mineral. Interestingly, contrary to the long help perception that intrafibrillar mineral requires either non-collagenous proteins or synthetic polymeric protein-analogues to form, Wang et al. demonstrated that intrafibrillar mineral can form in the absence of these acidic directing-agents, as long as the collagenous matrix has a high density that approximates that of natural bone or dentin . As a consequence of this well orchestrated process of biominerlization, a carbonated calcium-deficient hydroxyapatite mineral phase becomes the primary constituent of dentin. Approximately 50% by volume, 65% by weight (wet) of the tissue is composed of plate-like irregular hydroxyapatite (HAP) nanocrystallites (∼100 × 30 × 4 nm) . These are situated either within collagen fibrils (intrafibrillar) or between the fibrils, and (called either extra- or inter-fibrillar mineral) .
As mentioned above, it is generally accepted that nucleation of HAP nanocrystals begins in the gap zones of collagen fibrils and progresses via crystal growth and lengthening longitudinally between the intermolecular spaces separating collagen triple-helical molecules in a fibril (for a comprehensive description of the collagen hierarchical organization in dentin see Ref. ). This is relevant because it means that the specific size, thickness and alignment of the intrafibrillar mineral, are all guided (or physically restricted) by the adjacent collagen molecules, whereas the extrafibrillar mineral does not have such constraints; and thus the extrafibrillar mineral can assume more random orientations and have larger sizes . For instance, mineral in the collagen-free peritubular dentin has been shown to have a thickness of more than 10 nm . Conversely, Kinney et al. performed small angle X-ray scattering (SAXS) measurements on slices of dentine to conclude that the crystals in deep primary dentin are needle-like (unlike the more plate-like crystals found elsewhere), and have a thickness of ∼5 nm. Tesch et al. , on the other hand, used a more systematic analysis to show that the mean thickness of mineral platelets decreases from 3.6 nm to 2.3 nm in areas closer towards the pulp. These values are closer to the values presented more recently by Marten et al., who used small angle X-ray scattering to map 2D and 3D variations in mineral particle characteristics in entire molar crowns, and found a mean mineral platelet thickness of 3.2 nm decreasing to 2.6 nm farther beneath the dentin–enamel junction (DEJ), and becoming even thinner in deep dentine surrounding the pulp.
It is interesting to note that a major discrepancy in this field has been the lack of correlation in the intermolecular spaces within collagen fibrils – which is usually obtained from studies on non-mineralized collagen type I – and the size of mineral crystallites. For instance, it has long been acknowledged that the spaces separating the triple-helical molecules that compose a collagen fibril are in the range of approximately 2 nm. Nevertheless HAP crystals as large as 5 nm have been reported in the literature. To address some of the questions resulting for such discrepancies, Bonar et al. used neutron diffraction measurements of intact and demineralized hydrated bone, and determined the packing density of collagen molecules in mineralized bone collagen fibrils (which is representative of the space available for the mineral occupy) to be 1.24 nm, and this spacing increased considerably to 1.53 nm upon demineralization . The specific size of the mineral that is formed inside of the fibrils is of particular relevance for restorative treatments, especially adhesion to dentin, since dentin has a similar nanostructure to bone, and dental bonding depends on the putative penetration of adhesive monomers into collagen fibrils that have been demineralized . Hence the space available for monomer penetration is arguably dependent upon the thickness of the mineral crystallites that once occupied the intrafibrillar compartment. We discuss this aspect further in Section 3.1 below.
2.1
The importance of the intrafibrillar mineral
The presence and relevance of the intrafibrillar mineral in calcified tissues has been studied for many years. Early discussions surrounded the location and partitioning of the intrafibrillar mineral relative to the extrafibrillar component in these tissues . In the early 2000’s, work by Kinney at al was instrumental in providing insights into the specific contribution of the intrafibrillar mineral to the mechanical properties of dentin . Initially, high-resolution synchrotron radiation computed tomography (SRCT) and SAXS were used to show that dentin in teeth affected by dentinogenesis imperfecta type II (DI-II) had approximately 33% less mineral than healthy dentin. Most importantly, the DI-II dentin showed virtually no low-angle diffraction peaks corresponding to the presence of intrafibrillar mineral, consistent with the absence of intrafibrillar mineral .
In a subsequent report, DI-II dentin was used to study the mechanical contribution of the intrafibrillar mineral by comparing the nanoindentation elastic modulus of healthy dentin versus that of DI-II-affected dentin . Healthy dentin had a mean mineral volume of about 44.4%, whereas mineral volume in DI-II dentin varied from 30.9 to 40.5%. More surprisingly, the elastic modulus of dentin when measured in a hydrated state dropped drastically from 20 ± 1 GPa in healthy dentin down to only 5.7 ± 1.4 GPa in the DI-II dentin, despite only a moderate decrease in total mineral volume ( Fig. 1 ). These results have been interpreted on the basis of a simple rule of mixtures and the added shear stresses between mineral nanocrystals in intrafibrillar compartment , which is virtually lost in dentin lacking intrafibrillar mineral.

These early reports formed the basis for a series of contributions relative to the effective analyses of remineralized carious dentin. The conjecture that dentin lacking intrafibrillar mineral and having only a moderate decrease in mineral volume ratio caused a drastic decrease in mechanical properties begged the question whether simply determining mineral uptake by the tooth was a sufficient end point to determine successful remineralization. In 2009 a review paper published by our group summarized these questions , which together with other works describing the complexity of intrafibrillar remineralization in dentin provided impetus for a significant shift in the existing remineralization strategies and the evaluation of remineralized dentin. Accordingly, we showed that the nanoindentation modulus of dentin that was poorly remineralized could be as high 18 ± 2.7 GPa when measured in dry; however, when the same specimen was measured in wet conditions the elastic modulus dropped to only 1.6 ± 0.7 GPa, which is a drastic decrease of over 90% on average modulus ( Fig. 1 ). Two important interpretations resulted from these analyses: firstly it became apparent that mechanical properties of remineralized dentin measured in dry conditions might lead to false positive results; secondly, since hydrated dentin that lacked intrafibrillar mineral (DI-II dentin) had sharply lower modulus than healthy dentin – despite comparable mineral density (DI-II 40.5% vs. Healthy 44%) – it became apparent that effective dentin remineralization (determined on the basis of mechanical recovery) was only possible if both the extrafibrillar and intrafibrillar mineral components were replenished, ( Fig. 1 ) a conjecture that is largely supported today. Consequently, several strategies to remineralize dentin proposed to date highlight the importance of recovering the intrafibrillar mineral of demineralized (and carious) dentin (see Refs. for recent reviews), a process that has also been called functional remineralization, biomimetic remineralization, hierarchical remineralization and other similar terms. Similarly, these studies contributed to the development of numerous novel strategies that seek to prevent degradation of dentin-adhesive interfaces , of scaffold materials with improved biological and physical properties , and even the treatment of disease conditions such as DI-II-like dentin (Dspp knockout mice) . More importantly, these reports contributed to a significantly deeper understanding of the nanoscale and nanomechanical events that are now known to regulate the remineralization of dentin.
2
Dentin inorganic content—extrafibrillar and intrafibrillar mineral
During development, differentiated odontoblasts secrete the dentin matrix in a well orchestrated sequence that is characterized by the release of collagen fibrils with high concentrations of carboxylated non-collagenous proteins and proteoglycans . These matrix constituents, respectively, regulate the process of mineral deposition and fibrillogenesis during dentin formation. The process of collagen biomineralization has been a topic of much debate in the recent literature. Although it has long been acknowledged that collagen mineralization occurs due to mineral release from vesicles in the extracellular matrix (which have also been called calcospherites early on), it has recently been demonstrated that prior to secretion, the mineral accumulates intracellularly in the mitochondria , and travels to the extracellular space where it accumulates calcium while it remains in the form of amourphous calcium phosphate until full release onto the collagenous network .
Extensive work has shown that acidic non-collagenous proteins of the SIBLING family assist in stabilizing the calcium phosphate in the amorphous phase, aiding the penetration of the amorphous mineral in the gap zones and intermolecular spaces of collagen fibrils via capillary forces and osmotic-regulated mechanisms . Subsequent phase transformations allow the amorphous mineral to adopt a more crystalline and stable hydroxyapatite morphology , and form what is known as the intrafibrillar mineral. Interestingly, contrary to the long help perception that intrafibrillar mineral requires either non-collagenous proteins or synthetic polymeric protein-analogues to form, Wang et al. demonstrated that intrafibrillar mineral can form in the absence of these acidic directing-agents, as long as the collagenous matrix has a high density that approximates that of natural bone or dentin . As a consequence of this well orchestrated process of biominerlization, a carbonated calcium-deficient hydroxyapatite mineral phase becomes the primary constituent of dentin. Approximately 50% by volume, 65% by weight (wet) of the tissue is composed of plate-like irregular hydroxyapatite (HAP) nanocrystallites (∼100 × 30 × 4 nm) . These are situated either within collagen fibrils (intrafibrillar) or between the fibrils, and (called either extra- or inter-fibrillar mineral) .
As mentioned above, it is generally accepted that nucleation of HAP nanocrystals begins in the gap zones of collagen fibrils and progresses via crystal growth and lengthening longitudinally between the intermolecular spaces separating collagen triple-helical molecules in a fibril (for a comprehensive description of the collagen hierarchical organization in dentin see Ref. ). This is relevant because it means that the specific size, thickness and alignment of the intrafibrillar mineral, are all guided (or physically restricted) by the adjacent collagen molecules, whereas the extrafibrillar mineral does not have such constraints; and thus the extrafibrillar mineral can assume more random orientations and have larger sizes . For instance, mineral in the collagen-free peritubular dentin has been shown to have a thickness of more than 10 nm . Conversely, Kinney et al. performed small angle X-ray scattering (SAXS) measurements on slices of dentine to conclude that the crystals in deep primary dentin are needle-like (unlike the more plate-like crystals found elsewhere), and have a thickness of ∼5 nm. Tesch et al. , on the other hand, used a more systematic analysis to show that the mean thickness of mineral platelets decreases from 3.6 nm to 2.3 nm in areas closer towards the pulp. These values are closer to the values presented more recently by Marten et al., who used small angle X-ray scattering to map 2D and 3D variations in mineral particle characteristics in entire molar crowns, and found a mean mineral platelet thickness of 3.2 nm decreasing to 2.6 nm farther beneath the dentin–enamel junction (DEJ), and becoming even thinner in deep dentine surrounding the pulp.
It is interesting to note that a major discrepancy in this field has been the lack of correlation in the intermolecular spaces within collagen fibrils – which is usually obtained from studies on non-mineralized collagen type I – and the size of mineral crystallites. For instance, it has long been acknowledged that the spaces separating the triple-helical molecules that compose a collagen fibril are in the range of approximately 2 nm. Nevertheless HAP crystals as large as 5 nm have been reported in the literature. To address some of the questions resulting for such discrepancies, Bonar et al. used neutron diffraction measurements of intact and demineralized hydrated bone, and determined the packing density of collagen molecules in mineralized bone collagen fibrils (which is representative of the space available for the mineral occupy) to be 1.24 nm, and this spacing increased considerably to 1.53 nm upon demineralization . The specific size of the mineral that is formed inside of the fibrils is of particular relevance for restorative treatments, especially adhesion to dentin, since dentin has a similar nanostructure to bone, and dental bonding depends on the putative penetration of adhesive monomers into collagen fibrils that have been demineralized . Hence the space available for monomer penetration is arguably dependent upon the thickness of the mineral crystallites that once occupied the intrafibrillar compartment. We discuss this aspect further in Section 3.1 below.
2.1
The importance of the intrafibrillar mineral
The presence and relevance of the intrafibrillar mineral in calcified tissues has been studied for many years. Early discussions surrounded the location and partitioning of the intrafibrillar mineral relative to the extrafibrillar component in these tissues . In the early 2000’s, work by Kinney at al was instrumental in providing insights into the specific contribution of the intrafibrillar mineral to the mechanical properties of dentin . Initially, high-resolution synchrotron radiation computed tomography (SRCT) and SAXS were used to show that dentin in teeth affected by dentinogenesis imperfecta type II (DI-II) had approximately 33% less mineral than healthy dentin. Most importantly, the DI-II dentin showed virtually no low-angle diffraction peaks corresponding to the presence of intrafibrillar mineral, consistent with the absence of intrafibrillar mineral .
In a subsequent report, DI-II dentin was used to study the mechanical contribution of the intrafibrillar mineral by comparing the nanoindentation elastic modulus of healthy dentin versus that of DI-II-affected dentin . Healthy dentin had a mean mineral volume of about 44.4%, whereas mineral volume in DI-II dentin varied from 30.9 to 40.5%. More surprisingly, the elastic modulus of dentin when measured in a hydrated state dropped drastically from 20 ± 1 GPa in healthy dentin down to only 5.7 ± 1.4 GPa in the DI-II dentin, despite only a moderate decrease in total mineral volume ( Fig. 1 ). These results have been interpreted on the basis of a simple rule of mixtures and the added shear stresses between mineral nanocrystals in intrafibrillar compartment , which is virtually lost in dentin lacking intrafibrillar mineral.
These early reports formed the basis for a series of contributions relative to the effective analyses of remineralized carious dentin. The conjecture that dentin lacking intrafibrillar mineral and having only a moderate decrease in mineral volume ratio caused a drastic decrease in mechanical properties begged the question whether simply determining mineral uptake by the tooth was a sufficient end point to determine successful remineralization. In 2009 a review paper published by our group summarized these questions , which together with other works describing the complexity of intrafibrillar remineralization in dentin provided impetus for a significant shift in the existing remineralization strategies and the evaluation of remineralized dentin. Accordingly, we showed that the nanoindentation modulus of dentin that was poorly remineralized could be as high 18 ± 2.7 GPa when measured in dry; however, when the same specimen was measured in wet conditions the elastic modulus dropped to only 1.6 ± 0.7 GPa, which is a drastic decrease of over 90% on average modulus ( Fig. 1 ). Two important interpretations resulted from these analyses: firstly it became apparent that mechanical properties of remineralized dentin measured in dry conditions might lead to false positive results; secondly, since hydrated dentin that lacked intrafibrillar mineral (DI-II dentin) had sharply lower modulus than healthy dentin – despite comparable mineral density (DI-II 40.5% vs. Healthy 44%) – it became apparent that effective dentin remineralization (determined on the basis of mechanical recovery) was only possible if both the extrafibrillar and intrafibrillar mineral components were replenished, ( Fig. 1 ) a conjecture that is largely supported today. Consequently, several strategies to remineralize dentin proposed to date highlight the importance of recovering the intrafibrillar mineral of demineralized (and carious) dentin (see Refs. for recent reviews), a process that has also been called functional remineralization, biomimetic remineralization, hierarchical remineralization and other similar terms. Similarly, these studies contributed to the development of numerous novel strategies that seek to prevent degradation of dentin-adhesive interfaces , of scaffold materials with improved biological and physical properties , and even the treatment of disease conditions such as DI-II-like dentin (Dspp knockout mice) . More importantly, these reports contributed to a significantly deeper understanding of the nanoscale and nanomechanical events that are now known to regulate the remineralization of dentin.
3
Dentin organic matrix
3.1
Collagen
The hierarchical organization of collagen fibrils in dentin has been a topic of a recent a review by our group where we describe the structure and properties of collagen from the molecular to the micrometer length scale . Thus, here we will focus primarily in describing recent advances in the field in the past few years, as opposed to revisiting the structure of collagen in-depth.
Collagen represents one of the most intricate biological entities in the animal kingdom, where type I fibrillar collagen is also the most abundant protein in all species . Therefore, it is needless to say that research concerning the structure and properties of collagen surpasses the boundaries of dental research and dates back to several decades ago . Unfortunately, the complex interactions of dental materials with the molecular and nanostructure of dentin collagen, especially regarding the internal structure of individual fibrils, which is of tremendous relevance for dentistry, has only began to be explored in greater detail in recent years. For instance, many text books used to teach restorative and operative dentistry to date still characterize dentin collagen up to the fibrillar structural level , despite the fact that the foundation of modern restorative/adhesive dentistry based on the seminal work of Nakabayashi et al. relies on the proposed ‘enveloping and penetration’ of individual collagen fibrils with adhesive monomers. This invariably means that a good understanding of the internal structure of collagen is imperative to understand how monomers may interact with dentin at a fine scale. The requirement for such in-depth understanding has become even more relevant once the challenges of dentin nanoleakage have become increasingly evident in the literature .
Research has shown that dentin collagen has a typical diameter of approximately 80–100 nm , although other tissues have shown a much wider range of diameters . From larger to smaller structural features, collagen fibrils in dentin are essentially comprised of an array of laterally organized microfibrillar units, commonly referred to as collagen microfibrils . Recent work from our group suggests that these microfibrils may self organize in a ‘twisted’ array of bundles measuring approximately 20 nm each , therefore representing the second hierarchical level in dentin collagen within each of the 80–100 nm fibrils. However, these structures remain poorly characterized and their existence thus far has not been fully confirmed. Accordingly, these structures may also represent a transient state of collagen molecules that are more resistant to enzymatic degradation than others, as it has been reported for collagen in cartilage and tendon . Regardless, the next level of organization in dentin collagen is in the order of 5–6 nm in diameter, and relates to each individual collagen microfibril. Laterally, individual microfibrils are composed of 5 stranded tripled-helical collagen molecules that are organized in a quasi-hexagonal supramolecular supertwist . In other words, this means that each of the 5 triple-helical collagen molecules composing one microfibril is twisted around its own longitudinal axis and wrapped with 4 adjacent molecules in an arrangement that forms a near hexagonal shape. The molecules are staggered in a way that the repeating pattern of hundreds of them creates what is typically known as the D-periodical banding of collagen type I. Each triple-helical molecule has two alpha 1 and one alpha 2 chains intertwined via hydrogen bonds and electrostatic interactions along approximately 300 nm. Importantly, these individual chains are composed of a “coding system” (or recognition motifs) which are formed by the presence of specific amino acid sequences (X, Y, Gly), which ensure that each area of individual alpha chains can bind to adjacent chains, as long as the conditions for such recognition is adequate (i.e. collagen fibrillogenesis can occur at neutral pH despite the absence of cells or proteoglycans which are known to assist in the process of fibril formation) .
At the fibrillar level, several structures give rise to what may be described as collagen fibril surface nanostructural roughness. Gap and overlap zones form peaks and valleys at every 67 nm of each individual fibril. This repeated pattern (also known as collagen D-period) can interlock in a way that every valley (gap zone) is tightly adapted onto a peak (overlap zone) of an adjacent fibril , even when the collagen is hydrated. Each individual microfibril (5–6 nm) that composes a larger fibril (80–10 nm) can be visualized as longitudinal streaks on the surface of collagen fibrils via electron or atomic force microscopy ; and it is important to note that these structures are tightly bound with water molecules due to the inherent hydrophilic nature of collagen moieties. These nanometer scale ‘streaks’ may contribute to the formation of what has been called “nanovoids” during the steps of enveloping collagen with monomer molecules . It is important to note, also, that both dentin (at the tissue level) and collagen (at the molecular level) are inherently hydrated, and many monomers used in dentistry can undergo hydrolysis if kept in water for too long. Hence, such nanovoids can lead to the process of water tree formation that is typically seen in nanoleakege studies. Moreover, the pulpal pressure may facilitate diffusion of water molecules through these nanometer scale voids ( Fig. 2 ).
At the microfibrillar and molecular levels, several studies have attempted to dissect the specific separation distance between individual triple helical molecules composing individual microfibrils (which form a fibril) (see Ref. for a review). Interestingly, studies of the intermolecular space separating individual molecules in a fibril date back to the 70 s and earlier , and it has been well reported since then that not only these spaces are occupied by water molecules, but also that they are in the order of a few angstroms . The most recent model describing the molecular organization of collagen type I shows that the space between triple helical molecules averages at about 1.3 nm . That is to say that the space available for individual molecules to infiltrate is less than 2 nm.
In a recent review paper published by our group, we challenged the conjecture that such penetration was possible. That was based on the fact that (1) monomer molecules, for the most part, are delivered to dentin using high-density and relatively high-viscosity monomer blends (or low viscosity primers) that rely on diffusion alone to penetrate the collagen structure; and (2) we argued that the size of individual monomers might be too large to fit between the 1.3 nm intermolecular space within collagen fibrils. However, we failed to consider the wide range of motion that individual molecules can adopt in a given solvent, which may allow them to ‘squeeze’ into very tight spaces, such as within collagen fibrils. Work done by Takahashi et al. following our publication was instrumental in experimentally challenging the concept that we presented, and researchers were able to demonstrate that molecules typically used in common adhesive systems (i.e. HEMA and TEGDMA) could in fact penetrate the internal structure of dentin collagen, which is a very significant finding. Having said that, these experiments where performed with solubilized monomer molecules, as opposed to resin blends as used in adhesive systems, and in near ideal experimental conditions that are typically not found in the oral cavity. Additionally, it has been well documented that collagen molecules are individually wrapped by water forming cylinders of hydration ( Fig. 2 ), and although monomer molecules may infiltrate the internal structure of collagen it remains to be de determined whether or not the water in collagen is totally replaced by monomer molecules, which is quite unlikely. This internal hydration may be the reason for the hydrolytic degradation of the ester-containing monomers in the hybrid layer and the formation of the so-called water trees ( Fig. 2 ).
In summary, from a clinical stand point and taking into account the past decade in the literature, it is very well documented that nanoleakage is very common occurrence. Considering that (1) water molecules may degraded common dental monomers, (2) dentin and collagen are inherently hydrophilic and hydrated, (3) the pulpal pressure will invariability push water into the dentin-biomaterial interface, (4) the surface area of fibrils available for enveloping and infiltration is likely superior to the number of available monomer molecules to hermetically impregnate and wrap them, it comes to little surprise that the biomaterial/tooth interface remains the weakest link in restored teeth and that nanoleakege will continue to occur. Therefore, research should focus on not only overcoming these limitations, but also properly characterizing the interactions that weaken the dentin-biomaterial interface at the molecular and nanostructural length scales.
3.2
Non-collagenous components
Although dentin is primarily composed of mineral, collagen and water, it has been well documented that non-collagenous proteins, proteoglycans and several enzymes play a fundamental role in the homeostasis of the tissue. Each one of the individual non-collagenous entities in dentin has been a topic of focused reviews in recent years. The author is encouraged to refer to those papers, which have dealt with several aspects relative to the participation of MMPs in collagen/bonding degradation , of anionic carboxylated proteins in mineralization and development , and of proteoglycans in dentinogenesis . Here we will concentrate on the specific participation of key non-collagenous components in the biomechanics and structure of dentin and mineralized tissues of similar composition.
3.2.1
Proteins of the SIBLING family
The small integrin-binding ligand N-linked glycoprotein (SIBLING) family consists of osteopontin (OPN), bone sialoprotein (BSP), dentin matrix protein 1 (DMP1), dentin sialophosphoprotein (DSPP) and matrix extracellular phosphoglycoprotein (MEPE) . For a number of years these macromolecules have been characterized by their important participation in biomineralization during tissue development. Since these non-collagenous proteins constitute less than 5% of the dentin and bone matrices by volume, their participation in the structure and mechanical function at a tissue level have long been underestimated.
An important set of papers by Hansma’s group in the early 2000s used atomic force microscopy (AFM) force-spectroscopy to separate bone collagen fibrils and individual bone fragments to show that mineralized fibrils are interconnected with non-collagenous proteins that can be stretched, unfolded and refolded during tissue fracture . This mechanism was attributed to the presence of non-collagenous proteins of the SIBLING family in the interfibrillar region—although the involvement of proteoglycans was not ruled out. Thompson et al. and Fantner et al. , demonstrated that as collagen fibrils separate during tissue fracture, the ability of non-collagenous proteins to unfold before they fully rupture required the disruption of intramolecular bonds that hold the protein in a folded state, and as such bonds are broken, they dissipate significant levels of mechanical energy. More importantly, this mechanism can be repeated several times, every time that the collagenous network is subjected to stress at the nanometer scale, which is believed to increase tissue durability dramatically. These mechanisms gave rise to a concept where non-collagenous proteins began to be called the glue within our bones.
Importantly, work by Adams et al. , demonstrated that one of the non-collagenous proteins that has the ability of unfold to dissipate mechanical energy on the nanoscale is DMP1, which is found abundantly in dentin, and also in bone. Therefore, it is highly likely that similar mechanisms of mechanical energy dissipation are also present in dentin, and similar properties have also been described for inter-prismatic enamel proteins .
3.2.2
Proteoglycans
Similar to the SIBLING proteins described above, proteoglycans (PGs), which differ from other non-collagenous proteins in that they are (generally) constituted of a protein core covalently attached to a carbohydrate glycosaminoglycan (GAG) side chain , represent less than 3% of the dentin matrix by volume; and since their volume fraction is far less than those of collagen and apatite in mineralized tissues, their mechanical and structural participation has also been poorly explored for a number of years. This is an interesting conjecture, since PGs are known that play a fundamental role in the structural organization of the extracellular matrix (ECM) of soft tissues in all vertebrates.
PGs are believed to form interfibrillar supramolecular bridges between collagen in both mineralized and soft tissues . The GAG component of PGs is highly negatively charged, which allows GAGs to interact with one another between contiguous fibrils in an anti-parallel fashion (head-to-tail), forming tape-like aggregates that are further stabilized by electrostatic forces, H-bonds and hydrophobic interactions . Due to such a multi-level relationship, PGs and GAGs are known to absorb water and span the spaces between fibrils, which effectively signifies that the collagenous network is interconnected and held together by these non-collagenous structures on the nanoscale. Decorin (Dcn) and Biglycan (Bgn) are the two most abundant PGs in dentin whereas chondroitin-4-sulfate (C4S) and chondroitin-6-sulfate (C6S) appear as the most relevant GAGs in the matrix . Nevertheless, studies have also identified lumican, versican and trace amounts of fibromodulin in murine dentin .
The specific distribution of PGs and GAGs in dentin has recently been a topic of debate in the literature. A series of publications from Breschi’s group used gold labeling electron immunohistochemistry to show an increased presence of PGs and GAGs near the dentine tubule walls . This conjecture was further supported by recent studies in our lab where PGs appeared to form a membrane ( lamina limitans ) separating the intertubular from the peritubular dentin, while GAGs protruded out into the tubules forming an organic network that was not resistant to degradation with chondroitinase-ABC (C-ABC) ( Fig. 3 A–C) . Other recent studies determined the distribution of PGs and GAGs in carious dentin and demonstrated that tertiary dentin lacks these interfibrillar structures . However, their specific distribution of on a micro- to nanoscale remains unresolved. Recent preliminary work in our lab using second-generation harmonics microscopy, where the microscale structure and organization of the organic dentin matrix is visible without the requirement for demineralization, showed that microscale intertubular gaps or “holes” appear upon degradation of either GAGs, using C-ABC, or of the whole pool of non-collagenous proteins and PGs, using a trypsin enzyme ( Fig. 3 D–F). This is in agreement with earlier data by Hablitz et al. , where similar microscale intertubular gaps were imaged by AFM on the surface of fully demineralized dentin treated with a non-specific bleach solution. These observations point to the question of whether PGs and GAGs accumulate in higher concentrations in specific areas of the intertubular region of mineralized and mature dentin, as though they formed islands of concentrated PGs within the tissue, which remains to be tested more in depth.
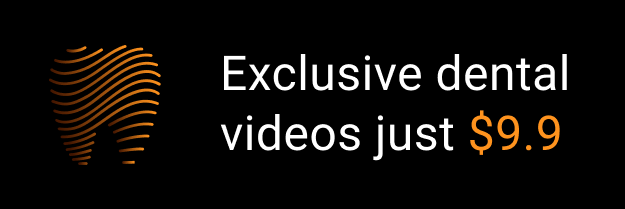