Fig. 5.1
Metabolic fate of sucrose. Sucrose can be metabolised to acids; energy is also generated during glycolysis which is needed for bacterial growth. Sucrose can also be converted to intracellular polymers (which can be catabolised to acid in the absence of dietary sugars) or extracellular polysaccharides (which contribute to the plaque matrix)
A matrix is a common feature of all biofilms and is biologically active, retaining water, nutrients and enzymes within the biofilm (Flemming and Wingender 2011; Koo et al. 2013). The chemistry of the matrix can also help exclude or restrict the penetration of other types of molecules, especially charged antimicrobial agents, while enabling some uncharged small molecules to penetrate deep into the biofilm. Gradients develop in factors that are critical to microbial growth, due to bacterial metabolism and diffusion restrictions, so that sites close together may be vastly different in the concentration of essential nutrients and toxic products of metabolism, as well as in terms of pH and oxygen tension, etc. Such vertical and horizontal stratification creates local environmental heterogeneity resulting in a mosaic of microhabitats or microenvironments and explains how organisms with apparently contradictory growth requirements (e.g. in terms of nutritional, atmospheric or pH requirements) coexist in plaque at the same site.
Multispecies biofilms create opportunities for numerous interactions among the constitutive species that are physically close to one another (Fig. 5.2). These include examples of conventional antagonistic and synergistic biochemical interactions, as well as gene transfer via plasmids and cell–cell signalling mediated by small diffusible molecules that enable similar bacteria to communicate with each other and coordinate their activities. Gram-positive bacteria communicate using small peptides, while both Gram-positive and Gram-negative species can use different molecules, such as autoinducer-2 (Jakubovics et al. 2014; Wright et al. 2013).
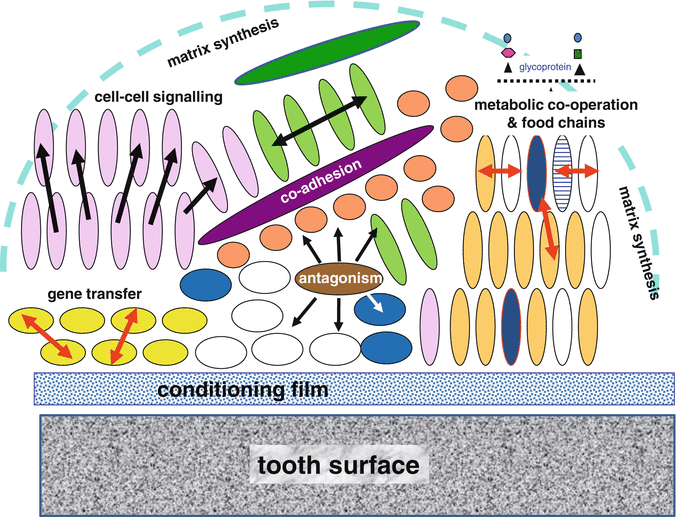
Fig. 5.2
A schematic representation of the interactions within a microbial biofilm such as dental plaque. The primary bacterial colonisers attach to the conditioning film (acquired pellicle) on the tooth surface by adhesin–receptor interactions; later, colonisers attach to the primary colonising bacteria (co-adhesion). As the biofilm develops, numerous interactions can occur among the component species. Synergistic interactions involve metabolic co-operation to catabolise endogenous nutrients (e.g. salivary glycoproteins), the development of food chains, cell–cell signalling, gene transfer and synthesis of an extracellular matrix. Antagonism can occur, for example, by the production of inhibitory molecules, which enable some species to outcompete others
As plaque matures, the microbiota becomes more diverse. A small sample of plaque may contain up to 100 distinct species, but the bacterial composition will vary at distinct anatomical sites due to differences in the prevailing biological conditions on each surface.
6.
Detachment from surfaces. Bacteria may be able to ‘sense’ adverse changes in environmental conditions, and these may act as cues to induce the genes involved in active detachment.
5.6 Microbiology of Caries
Despite dental biofilms being natural and contributing to the well-being of the host, on occasions, this symbiotic relationship can break down and disease occurs (dysbiosis) (Marsh et al. 2015b).
Fissures are the most caries-prone sites of the dentition. Early cross-sectional and longitudinal studies of fissures reported a strong relationship between increased proportions of mutans streptococci (mainly Streptococcus mutans and S. sobrinus) in the biofilm and the detection of a caries lesion (Loesche 1986). However, this relationship was not absolute, and there were lesions from which these organisms could not be detected in the overlying biofilm and cases of mutans streptococci persisting in the absence of a lesion.
Similarly, early cross-sectional studies of approximal surfaces found a positive correlation between elevated mutans streptococci levels and lesion development, although a less clear-cut association was found in some longitudinal studies (Marsh and Martin 2009; Marsh et al. 2015b). Again, at some sites, mutans streptococci could be found in high numbers before the radiographic detection of demineralisation, while some lesions developed in the apparent absence of these bacteria. Mutans streptococci could also be present at some sites for prolonged periods in high numbers without any evidence of caries.
When appropriate culture media have been used, recent studies have found associations between other groups of bacteria and caries, especially bifidobacteria (Mantzourani et al. 2009a, b).
Contemporary studies of dental biofilms now use either culture-independent molecular approaches or a combination of culture and molecular techniques to analyse the microbiota. A recent study of severe early childhood caries of different tooth surfaces at various stages of caries formation using a combination of anaerobic culture and molecular-based techniques found that disease was associated with a diverse microbiota, including some previously uncultivated species. The species most closely associated with this severe form of caries included S. mutans, Scardovia wiggsiae, Veillonella parvula, Streptococcus cristatus and Actinomyces gerencseriae (Tanner et al. 2011). Similar findings had been reported in a previous study of early childhood caries using culture-independent approaches but with the limitation that samples of dental biofilms had been pooled prior to analysis. S. sanguinis was associated with sound enamel, while A. gerencseriae, Bifidobacterium spp., S. mutans, S. salivarius, S. constellatus, S. parasanguinis, L. fermentum and Veillonella spp. were associated with caries (Becker et al. 2002). The data suggested that some Actinomyces spp. may be significant in caries initiation while Bifidobacterium spp. may play a role in more advanced lesions. Likewise, in a molecular-based study of pooled plaque of caries in primary and permanent teeth in children, a number of species in addition to S. mutans were shown to play a role in caries progression; these bacteria included members of the genera Veillonella, Lactobacillus, Bifidobacterium, Propionibacterium, Actinomyces and Atopobium, plus low-pH non-S. mutans streptococci (Aas et al. 2008).
Collectively, the data from numerous surveys of various tooth surfaces, of different patient age groups from numerous countries and populations with different dietary habits, etc., have shown a strong positive association between increased levels of mutans streptococci and the initiation of demineralisation. However, not every study identified all of the bacteria present in the clinical samples, and some focussed only on organisms already implicated in disease, e.g. mutans streptococci and lactobacilli.
While several bacterial species may contribute to demineralisation, a number of others may reduce the impact of acid production either by utilising the lactate produced from sugar metabolism (e.g. Veillonella spp.) or by producing alkali from saliva components (S. salivarius, S. sanguinis, A. naeslundii) (Marsh et al. 2015b). Thus, caries is a complex microbiological process as it is the outcome of a number of interactions between acid-producing and acid-utilising or acid-neutralising species in a structurally organised biofilm.
5.7 Cariogenic Features of Dental Biofilm Bacteria
In order for bacteria to play a role in caries, they must possess certain caries-promoting characteristics (Marsh et al. 2015b), which centre around the metabolism of fermentable dietary sugars, especially sucrose (Fig. 5.1), and include:
-
The ability to rapidly transport fermentable sugars when competing with other plaque bacteria and the conversion of such sugars to acid and the generation of a low pH. Most saccharolytic bacteria in dental plaque, including mutans streptococci, possess several sugar transport systems, including high-affinity phosphoenolpyruvate: sugar phosphotransferase (PEP-PTS) systems, which are able to scavenge sugars even when they are present in the oral environment only in low concentrations. These sugars are converted to acids (and predominantly lactic acid) by glycolysis; caries-associated bacteria can generate a low pH (e.g. less than pH 5.0) in a few minutes.
-
The ability to maintain sugar metabolism and remain viable under extreme environmental conditions, such as at a low pH. Many plaque bacteria can make acid from sugar metabolism, but far fewer are able to tolerate acidic conditions for prolonged periods. Mutans streptococci, lactobacilli and bifidobacteria not only remain viable at a low pH but manage to continue to grow and metabolise, i.e. they are both acidogenic and aciduric (acid tolerant). This ability relies on a series of biochemical properties: (i) the activity of proton-translocating ATPase (proton exclusion inside to outside of bacterial cells); (ii) alkali production, due to the activity of bacterial ureases, arginine deiminase and other alkali-producing systems; (iii) proton impermeability of bacterial cell membrane; and (iv) the production of stress proteins (protection of cellular structural and functional proteins from acid denaturation) (Takahashi and Yamada 1999).
-
The production of extracellular (EPS) and intracellular polysaccharides (IPS). EPS include glucans and fructans, both of which contribute to the biofilm matrix. IPS are glycogen-like storage compounds that can be used for energy production and converted to acid when free sugars are not available in the mouth.
Mutans streptococci are known to have all these properties (Table 5.1) and are well equipped to act as cariogenic bacteria (Loesche 1986). However, these properties are not specific to mutans streptococci in the same way that the possession of some virulence factors (e.g. cholera toxin for Vibrio cholerae, pertussis toxin for Bordetella pertussis) helps to define certain classical medical pathogens. Indeed, the acidogenic and aciduric profile of bacterial species lies along a continuum, with evidence of considerable overlap (Table 5.1). Some strains of non-mutans streptococcal species, e.g. S. mitis, S. gordonii, S. anginosus and S. oralis, can be as acidogenic and acid tolerating as some examples of mutans streptococci (de Soet et al. 2000; van Houte 1994; van Ruyven et al. 2000). Therefore, it is not surprising that there is a lack of total specificity in the microbial aetiology of dental caries.
Table 5.1
Cariogenic characteristics of some oral bacteria
Bacterium
|
Cariogenic property
|
||||
---|---|---|---|---|---|
Sugar transport (rate)
|
Acid production (rate)
|
Acid tolerance
|
Polymer production
|
||
Extracellular
|
Intracellular
|
||||
Mutans streptococcia
|
Multiple systems
(Very rapid)
|
<pH 4.0
(Very rapid)
|
High
Grows at pH 5.5 and below
|
Soluble and insoluble polysaccharides (glucans and fructan)
|
Yesb
|
Lactobacillic
|
Little known of systems
(Rapid)
|
<pH 4.0
(Rapid)
|
Very high
Growth at pH 5.0
|
Heteropolysaccharide
Glucan
|
No
|
Actinomyces speciesd
|
Little known of systems
![]() Stay updated, free dental videos. Join our Telegram channel![]() VIDEdental - Online dental courses![]() ![]() ![]() |