Abstract
Objective
To measure the degrees of conversion (DC), conversion kinetics, and the effect of post-irradiation time on rapid photo-polymerized bulk-fill resin composites under conditions equivalent to clinical depths of 1 and 4 mm.
Methods
36 specimens (n = 3), based on two resin composites incorporating PowerCure rapid-polymerization technology in two consistencies (PFill; PFlow) and two comparators with matching consistencies (Eceram; EFlow), were investigated from the same manufacturer (Ivoclar AG, Liechtenstein). Specimens were prepared within 4 mm diameter cylindrical molds, of either 1 mm or 4 mm depths respectively, to simulate near-surface and deep locations in a bulk-fill restoration. The independent variables in this study were: materials, thickness and time. Two high irradiance polymerization protocols were utilized for PowerCure materials: 2000 and 3050 mW/cm 2 for 5 and 3 s, respectively. A standard (1200 mW/cm 2 ) polymerization protocol was used with control materials. FTIR was utilized to measure DC in real-time for 24 h post-irradiation. The data were analyzed using Welch’s -ANOVA, Games-Howell post-hoc test , kinetic dual-exponential sum function and independent sample t- tests (p = 0.05).
Results
The DC of the materials ranged between 44.7–59.0 % after 5 min, which increased after 24 h reaching 55.7–71.0 % (p < 0.05). Specimen thickness did not influence the overall DC. At 5 min, the highest DC was shown in EFlow. But PFlow, irradiated for 3 s and 5 s exhibited comparable results (p > 0.05). PFill composite irradiated with the 3 s and 5 s protocols did not differ from ECeram (p > 0.05). Specimen thickness and material viscosity affected polymerization kinetics and rate of polymerization (RP max ). Faster polymerization occurred in 1 mm specimens (except PFill-5 s and ECeram). PFill and PFlow exhibited faster conversion than the controls. RP max varied across the specimen groups between 4.3–8.8 %/s with corresponding DC RPmax between 22.2–45.3 %.
Significance
Polymerization kinetics and RP max were influenced by specimen thickness and material viscosity. PFill and PFlow materials produced an overall comparable conversion at 5 min and 24 h post-irradiation, despite the ultra-short irradiation times, throughout the 4 mm specimen thickness.
1
Introduction
A representative general dentist spends more than half of their clinical time placing direct restorations, most of which are resin composites [ ]. These have become the preferred type of direct restorative materials owing to the recent advancements in their properties. Furthermore, dentists are always looking for an efficient way to manage their practice including fast set materials and shortened clinical steps to reduce overall expenses.
This was endorsed by composite manufacturers via recent facilitation in resin composite application, with less clinical steps and shorter photo-polymerization time. However, this requires operators to develop increased understanding of their materials and their applications [ ]. Even though the polymerization reaction is essentially chemical, operators still control some key aspects of the reaction. The radiant energy and exposure time determine the amount of energy delivered to excite the photoinitiators and thus the rate and quality of polymerization [ , ]. Also, the selected filler load, shade, increment thickness, distance and angulation of light-curing tip are all operator-related factors that affect material behavior upon polymerization and future properties [ ]. Sub-optimal delivery of energy can cause low degree of conversion and physical instability leading to further complications such as marginal deterioration, recurrent caries, and bulk fracture [ ].
Photo-polymerization of resin composite begins as light propagates through the material depth. Photon energy activates the free radical generators (photoinitiators). Depending on the photoinitiator type (Norrish type I, II), these photoinitiators will generate free radicals seeking another electron to bond with. Methacrylate groups, in most dental monomers, will instantly bond to the free radical, at one end of the (C C) double bond. The other end will become a radical and the reaction will continue to propagate. This chain reaction will auto-accelerate rapidly, until the concentration of the available monomers diminishes and/or the densification process limits the monomer movements and hinders further reaction [ ]. Random rapid crosslinking can ultimately reduce molecular movements, resulting in a nonhomogeneous network and potentially a less-than-optimal DC. This can lead to future elution of unreacted monomers, causing compromised physical stability and mechanical properties [ ].
One approach to improve the physical stability of the resin composites is by controlling the polymer network architecture through the radical polymerization. The idea is that by modifying the radical chain growth to a step-like polymerization, a more homogenous polymer network with improved physical and mechanical properties will be achieved. A reversible addition-fragmentation chain transfer (RAFT) polymerization mechanism, by the addition of β-allyl sulfone (AFCT) reagent, was proposed previously to be incorporated in dental polymers [ ]. Incorporating (AFCT) reagent to a dimethacrylate network has been shown to improve the network architecture during polymerization leading to enhanced network homogeneity, thermal glass transition temperature, and mechanical properties [ , ]. This promising modification was utilized in a bulkfill resin composite materials as part of a restorative system, PowerCure, (PowerFill and PowerFlow; Ivoclar Vivadent AG, Liechtenstein). These restorative materials were designed to work with high irradiance (3050 ± 10 % mW/cm 2 ) received from a polywave light-curing unit (LCU: Bluephase PowerCure).
The efficacy of polymerization may decrease in deep layers due to light attenuation in 2−3 mm depth. A reduced number of photons reaching the bottom layer means fewer activated photoinitiator molecules [ , ]. Therefore, the bottom layer DC is important to understand polymerization-related properties. Fourier transform infrared (FTIR) spectroscopy is routinely used to measure DC, with mid-IR spectrum ranges between 4000−400 cm −1 which interacts with molecular vibration modes [ , ]. Following irradiation, polymerization may continue for more than 7 days post-irradiation [ ]. However, 24 h post irradiation measurements are sufficient to determine polymer matrix formation [ , ].
The effect of using such high irradiance light-curing units is still not fully explored. Therefore, the aim of this study was to evaluate the degree of conversion (DC), conversion kinetics, and the effect of 24 h post-irradiation time on rapid photo-polymerized resin composites at depths-within-specimens of 1 and 4 mm, relative to the irradiated surface. The null hypotheses were that: there are no differences in the DC between rapid photo-polymerized resin composites and conventional photo-polymerized comparator composites: (a) at 1 and 4 mm depths; and (b) for 5 min and 24 h post-irradiation times.
2
Materials and methods
2.1
Study design
The independent variables in this study were: materials, thickness, and time. Two resin composite materials incorporating rapid-polymerization technology and two comparators were investigated. Data on the resin composite compositions were obtained from the manufacturer ( Table 1 , Fig. 1 ). PowerCure materials are available in flowable (lower viscosity) and non-flowable (regular viscosity) consistencies. Two comparator materials were studied with matching consistencies. The purpose of this study was to investigate the PowerCure materials within their system. Therefore, two high irradiance light protocols were utilized for the PowerCure materials: 2000 mW/cm 2 for 5 s and 3050 mW/cm 2 for 3 s, respectively. A standard (1200 mW/cm 2 ) light curing unit (LCU) was used with the control materials ( Table 2 ). The radiant emittances were all verified with a calibrated MARC™-LC instrument (BlueLight Analytics, Halifax, Ca).
Material code | Product name | Lot number | Resin matrix a | Filler load % (wt);(vol) |
---|---|---|---|---|
PFill | Tetric PowerFill | W92823 | Bis-GMA, Bis-EMA, UDMA, Aromatic Dimethacrylate, DCP | 79 %; NA |
PFlow | Tetric PowerFlow | WM1175 | 71%; NA | |
ECeram | Tetric EvoCeram Bulk Fill | U53769 | Bis-GMA, Bis-EMA and UDMA | 80; 61 % |
EFlow | Tetric EvoFlow Bulk Fill | U42390 | Bis-GMA, Bis-EMA, TCDD | 68.2; 46.4 % |
a Matrix monomer: Bis-GMA: bisphenol-A-diglycidyl dimethacrylate; Bis-EMA: bisphenol-A-polyethylene-glycol-diether dimethacrylate; UDMA: urethane dimethacrylate.

Light-curing unit | Emission spectral type | Protocol | Time | Irradiance mW/cm 2 |
Radiant exposure J/cm 2 | Manufacturer |
---|---|---|---|---|---|---|
Bluephase PowerCure (P-Cure) |
wide spectrum | 3 s | 3 s | 3000 | 9 | Ivoclar Vivadent AG, Liechtenstein |
Turbo | 5 s | 2000 | 10 | |||
Elipar S10 (S10) |
narrow spectrum | Standard | 20 s | 1200 | 24 | 3M ESPE, MN, USA |
2.2
Specimen preparation
Specimens were prepared within 4 mm cylindrical molds of either 1 or 4 mm depth (thickness) to simulate, respectively, near-surface and deep locations in bulk-fill restorations. All specimens were fabricated and measured at 23 ± 1 °C. Twelve specimen groups (n = 3) were created for each permutation of material /irradiation protocol and the two measurement depths [Tables: 1, 2, 4]. The sample size was confirmed using G*power software (V. 3.1.3; Heinrich Heine University, Germany) based on a pilot study. A sample size: n = 3 was sufficient to give a power over 80 %.
2.3
Degree of conversion
The DC of the materials was measured by a Fourier-transform infrared (FTIR) spectrometer (ALPHA II, Bruker, Massachusetts, USA), with a single reflection ATR accessory. Uncured composite materials, within 1 or 4 mm deep molds, were placed over the ATR crystal ( Fig. 2 ). The spectrum of each uncured material was recorded for the duration of two scans (10 s). The specimens were then irradiated using the designated LCU, for specified periods ( Table 2 ). Using OPUS software (BRUKER OPTIK GmbH, Ettlingen, Germany), FTIR spectra were recorded in real-time for 24 h post-irradiation. That is, each specimen remained in position at 23 °C on the ATR crystal for 24 h, while spectra were recorded continuously. Subsequently, from the resultant datasets, DC (%) values at selected relevant time intervals (5 s, 60 s, 5 min, 24 h) were plotted as a function of Log 10 time (s), ( Fig. 3 ). The parameters were as follows: 4000−400 cm −1 wavelength; 4 cm −1 resolution. The instrument was calibrated daily by generating a background spectrum to be implemented in the specimen’s spectrum.


To calculate DC, the variation in peak height ratio using the two-frequency technique (C C stretch at 1637 cm −1 against an internal reference frequency at 1608 cm −1 ) was utilized. The per-cent degree of conversion, from Eq. (1) , was calculated and plotted over time.
DC%=1−1637cm−11608cm−1peakheightsafterpolymerization1637cm−11608cm−1peakheightsbeforepolymerizationX100
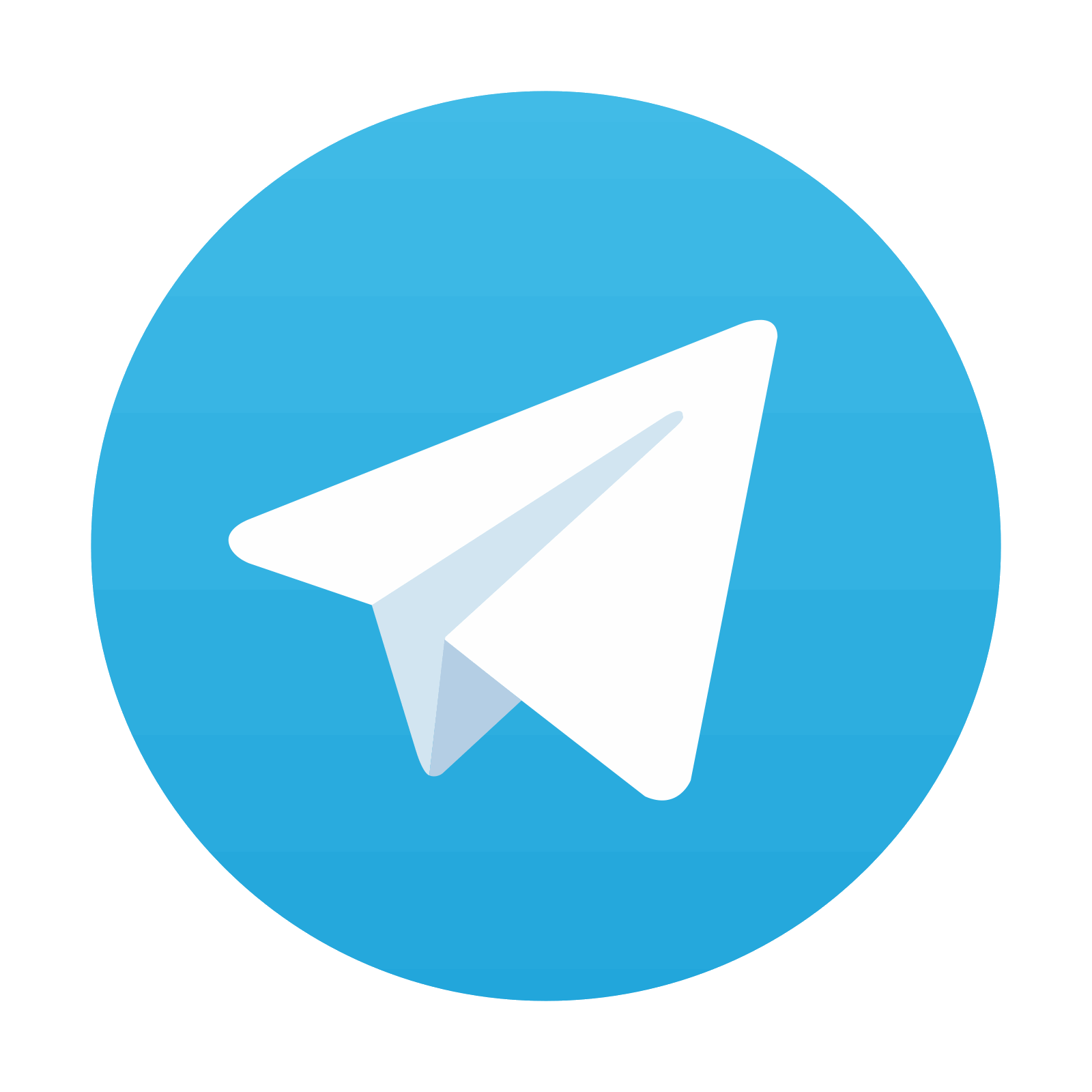
Stay updated, free dental videos. Join our Telegram channel

VIDEdental - Online dental courses
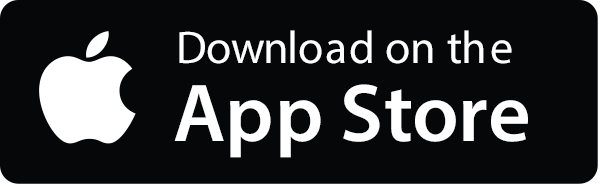
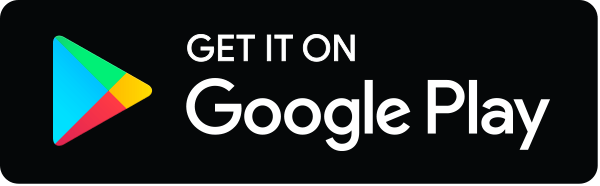
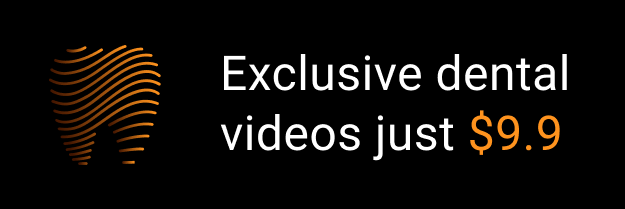