Graphical abstract

Highlights
- •
Pore size and GO wrinkle degree were elevated with the growing content of CF.
- •
Porous layer provided an accessible channel for nutrients transport for cell growth.
- •
GO wrinkle promoted osseointegration in vitro and in vivo dramatically.
- •
The porous layer and GO wrinkle showed no cytotoxicity and systemic toxicity.
Abstract
Objectives
To investigate the influence of surface microstructure and chemistry after modification on surface bioactivity and biosafety of carbon fibers reinforced PEEK (CF/PEEK) composites as implants.
Methods
CF/PEEK composites with different CF contents (0 wt%, 25 wt% and 40 wt%) were prepared by injection molding and treated by concentrated sulfuric acid. A porous network was produced on the surface by etching action. Subsequently, the sulfonated CF/PEEK composites were immersed in GO solution. Thus, GO wrinkles with abundant functional groups were wrapped outside the porous nanostructures on CF/PEEK composites. The cell responses in vitro (proliferation, alkaline phosphatase activity and cell mineralization), osseointegration in vivo (fluorochrome labeling, H&E staining and X-ray analysis) and biosafety were investigated.
Results
The pore size of porous layer on the surface of CF/PEEK composites was improved with the increase of CF content. Subsequently, a silk-like GO wrinkles on the surface were formed by GO modification. And the more CF content, the greater the degree of GO wrinkles. The results revealed that GO functional wrinkle up-regulated surface hydrophilicity. In vitro cell experiments showed that porous nanostructures and GO wrinkles dramatically promoted initial cell behaviors. Significantly, GO modified composites exhibited enhanced bioactivity and osseointegration in vivo. Fortunately, the GO wrapped porous CF/PEEK composites displayed biosafety.
Significance
The surface modification is effective and the modified composites showed great bioactivity. The GO wrapped porous CF/PEEK composites would hold great potential for implants.
1
Introduction
In recent years, Polyether ether ketone (PEEK) and PEEK composites have been considered as acclaimed promising implant materials since proper mechanical properties (especially elastic modulus) compared to typical metallic materials, such as titanium and its alloys [ ]. The elastic modulus of titanium (over 100 GPa) mismatches that of human bones (about 18–25 GPa), which may cause bone absorption [ ]. PEEK has been proved to have elastic modulus (3–4 GPa) close to that of natural human bone, which may reduce the concerns of bone absorption and osteoporosis caused by stress shielding [ ]. Furthermore, PEEK and PEEK composites show well-known biocompatibility, natural radiolucency and chemical resistance [ , ]. In spite of these excellent attributes, the main defects hindering their implantable applications are the inert surface and poor osteogenesis [ , ].
Osteogenic differentiation involves a series of complex biological events, such as protein adsorption, blood clotting, site infiltration as well as surface biological recognition between mesenchymal stem cells (MSCs) [ ]. These steps are directly and indirectly influenced by the surface geometric structure and chemical composition of implants [ ]. It is generally believed that the surface geometry of the material can directly regulate the hydrophilicity and roughness of the surface, which synergistically affects initial cellular behavior and long-term osseointegration [ ]. Some studies have confirmed that porous biomaterials showed higher surface bioactivity. Many ideas have been proposed to create porous structures on the surface of PEEK implants. Yuan et al. [ ] proved that chemical treatment with suitable H 2 SO 4 created porous sulfonated layers on the surface of PEEK, which was conducive to improve surface bioactivity. Zhao et al. [ ] indicated that sulfonated porous PEEK exhibited dramatically improved bioactivity and osseointegration both in vitro and in vivo. In addition to the porous structure provides a suitable roughness for cell growth, it also tends to encourage ingrowth of soft and hard tissue into the implants, which would bring strong biological anchorage to enhance the stability of the implants [ ]. Furthermore, the porous structure on implant surface could generate favorable spaces and channels for nutrients transportation [ ]. Therefore, sulfonation is a simple and effective strategy for PEEK surface treatment with little damage to mechanical properties [ ].
Although the sulfonated PEEK shows great surface biological activity, it cannot be ignored that its low mechanical strength and elastic modulus is still a disadvantage that restricts its application for particular indications in dentistry [ ]. Recent years, carbon fibers (CF) with different contents have been added into PEEK to improve the mechanical properties. The mechanical strength and elastic modulus of PEEK can be effectively improved by adding CF. More attractively, the mechanical strength can be adjusted so as to better adapt to the different demands of human skeleton on the endurance of force by adjusting the content of CF [ ]. Many studies have been carried out on sulfonation treatment of PEEK, but the effect of sulfonation treatment on the surface morphology, cellular response in vitro, osseointegration in vivo and biosafety of CF/PEEK composites are seldom studied. So the effects of CF addition and CF content on the porous structures formed by sulfonation need to be further explored.
As biomaterials, biosafety (cytotoxicity and systemic toxicity) should also be noticed to ensure the implant will not cause inflammation and pathological changes in the adjacent tissues and important organs of whole body. Although the sulfonated porous structures is conducive to bioactivity, a large number of sulfur-containing groups have been considered to have a negative impact on human cells and tissues [ ]. Low-valent sulfur produces oxygen free radicals will damage cell structures, moreover, sulfur dioxide and its derivatives could cause DNA damage. Therefore, how to limit the dissociation of sulfonic acid groups in body fluid is very important. Graphene oxide (GO) as a two-dimensional new carbon material, has been considered as an attractive bioactive material because of its unique lamellar structure, large specific surface area, sp 2 carbon domains and abundant oxygen-containing function groups (hydroxyl, carboxyl, etc.) on its surface [ , ]. These functional groups make GO easy to realize chemical bonding with other substances. So, it is considered the dissociation of sulfonic acid groups could be limited by the encapsulation and chemical action of GO coating, to ensure the biological safety of implants. Furthermore, some study revealed that GO was able to facilitate attachment, proliferation and osteogenic differentiation of bone marrow mesenchymal stem cells (BMSCs) due to the cellular behaviors and protein adsorption could be regulated by the abundant hydrophilic functional groups on GO [ , ]. Considering the remarkable bioactivity properties, GO could be a promising functional modification material to modify the surface of CF/PEEK composites after sulfonation.
In this work, we hypothesized that altering surface properties by combining sulfonation and GO modification might be a feasible strategy to improve bioactivity of CF/PEEK implants. Our previous work confirmed that CF/PEEK composites with 25 wt% and 40 wt% carbon fiber could effectively improve the mechanical properties of composites to meet the needs of human bones [ ]. Lack of CF will cause insufficient mechanical strengthening, while overloading CF (CF content over 40 wt%) may lead to the failure of injection molding due to the high viscoelasticity of CF/PEEK mixture. We developed and characterized GO modified sulfonated CF/PEEK composites with 25 wt% and 40 wt% carbon fibers. The objectives of this work are (1) to assess in vitro how bone marrow mesenchymal stem cells (BMSCs) responded on the modified CF/PEEK surfaces in terms of proliferation, alkaline phosphatase (ALP) activity and cell mineralization; (2) to investigate in vivo osteogenesis ability of bony tissue exposed to the modified composites in calvarial defect models; (3) to evaluate biosafety of the developed implants in vitro (cytotoxicity) and in vivo (histopathological assess). This work could expedite the pace of PEEK based materials to orthopedic/dental implants.
2
Materials and methods
The whole experimental process is concisely summarized in Fig. 1 . CF/PEEK composites with different CF contents were successfully prepared by injection molding. Then the surfaces of PEEK and CF/PEEK composites were sulfonated by concentrated sulfuric acid. Afterwards, CF/PEEK was immersed in GO aqueous solution to obtain GO surface modified CF/PEEK. Meanwhile, untreated CF/PEEK was set as a control group. The surface physicochemical properties were tested to explore the effects of surface modification on surface structure and chemistry. Moreover, in vitro cell behavior and in vivo animal implantation were investigated to evaluate the effects of surface modification on osteogenesis and biosafety of CF/PEEK composites.

2.1
Fabrication and characterization of surface modified CF/PEEK composites
2.1.1
The preparation of CF/PEEK composites
To improve the dispersion of carbon fibers in PEEK, a twin screw extruder (SJZS-10A, China) was used to mix CF and PEEK. Then, CF/PEEK composites were prepared by injection molding (SZS-20, China) at 380 °C. Different CF contents in the composites were set including 25 wt% and 40 wt% (named 25CF and 40CF, respectively) meanwhile pure PEEK without CF was prepared as control (0CF). Then all the CF/PEEK composites were tailored into square pieces (10 mm × 10 mm) for in vitro studies. Disks of CF/PEEK composites (5 mm of diameter, 1 mm of thickness) were used for in vivo studies. All the samples were polished with abrasive paper and fully cleaned in acetone, ethanol and deionized water with ultrasonic for 15 min and air dried in oven (at 60 °C) for the surface modification.
2.1.2
Sulfonation and GO modification of materials
The concentrated sulfuric acid (95–98 wt%) was applied to do surface sulfonation. The specific approach was as follows. First, samples were soaked in concentrated sulfuric acid for 10 min. After that, the sulfonated samples were taken out and washed slowly with deionized water. Then the sulfonated samples were bathed in deionized water for 10 min. Afterwards, they were immersed in the acetone for another 10 min. Finally, the sulfonated PEEK and CF/PEEK composites were obtained after dried for 30 min in oven (at 60 °C). After the sulfonation, 0CF, 25CF and 40CF were renamed to S-0CF, S-25CF and S-40CF.
The samples were then modified by GO. Our group has rich experiences in preparation and characterization of GO [ ]. GO was prepared by Hummers method as before [ ] and it was characterized in our previous work [ ]. Specifically, 30 mg of GO were dispersed in 30 mL of deionized water. Then the samples were immersed in the GO solution for 10 min. Next, the samples were dried in oven at 100 °C for 10 min. Again, the samples were immersed in the GO solution for 10 min and dried for 10 min. Repeat the above steps for five times. Finally, GO modified samples were washed with ultrasonication (40 kHz) in acetone, ethyl alcohol and deionized water for 5 min, respectively. Then, the modified samples were dried at 100 °C overnight. After the treatment by GO, S-0CF, S-25CF and S-40CF were renamed to GO-S-0CF, GO-S-25CF and GO-S-40CF.
2.1.3
Surface characterization
Fourier transform infrared (FTIR) spectrophotometer equipped with an attenuated total reflection (ATR) was used to measure spectra of the samples at room temperature. All spectra were collected from 600 to 2000 cm −1 at a resolution of 2 cm −1 by 32 scans in total.
Scanning electron microscope (SEM, MIRA3LMH, TESCAN, a.s.) equipped with an energy dispersive spectrometry (EDS) was used to observe the surface morphology of the surface treated PEEK and CF/PEEK composites. Before observation, samples were coated with gold for 60 s.
Surface roughness was measured by laser confocal microscopy (VK-X200K, KEYENCE, Japan) at 200 times magnification (25 °C). The roughness measurement parameters were set as: RMR (−23 μm); Rδc (standard MR: 25%; comparative MR: 75%); Rmr (c) (26 μm, 50%). Three parallel samples were tested (n = 3).
According to ISO 15989-2004, contact angle was measured by a Contact Angle Meter (Solon Tech. (Shang Hai) Co., Ltd., SL200B) at 23 ± 2 °C and the volume of the water drop was 2 μL. Six parallel samples were tested in this work (n = 6).
2.2
Cell attachment, proliferation, ALP activity and mineralization potential of BMSCs on the modified surfaces in vitro
2.2.1
Solation and culture of BMSCs
Bone marrow mesenchymal stem cells (BMSCs) isolated from male Sprague Dawley (SD) rats were used to detect the bioactivity of the samples. All animal experiments were approved by Medical Ethics Committee of Shanxi Medical University and executed in compliance with the US National Institutes of Health Guidelines on the Care and Use of Laboratory Animals. 120 g of male Sprague Dawley (SD) rats were purchased from the Animal Experimental Center of Shanxi Medical University. After anesthesia, the rats were sacrificed and immersed in 75% ethanol (ANNJET, Shandong) for 5 min. Bilateral femur and tibia were separated, disinfected with ethanol and cleaned with PBS (BOSTER, Wuhan) for three times. The bone marrow cavity was exposed by scissors and the marrow cavity was repeatedly and gently rinsed with ɑ-MEM (BOSTER, Wuhan). Afterwards, the non-adherent cells were removed after 48 h of culture in 37 °C and 5% CO 2 incubator. Then, the culture medium was replaced every 2 days. Finally, the cultured bone marrow mesenchymal stem cells (BMSCs) in passage 3–5 (P3 – P5) were used in subsequent experiments.
2.2.2
Cell attachment and numbers of adhered BMSCs on the composites
BMSCs were inoculated on samples in 24-well plate (n = 3) with a cell density of 5 × 10 4 cells/mL. After cultured in 37 °C and 5% CO 2 incubator for 48 h, samples were rinsed twice with PBS. After immobilization and permeation, immunofluorescence staining was performed with TRITC Phalloidin Rhodamine labeled phalloidin working fluid (Yeasen Biotech Co., Ltd., Shanghai) and DAPI dye solution (BOSTER, Wuhan). Lastly, stained cells were observed and the numbers were counted under confocal laser microscopy (Olympus, Japan) (n = 3).
2.2.3
BMSCs proliferation on the composites
Cell proliferation of BMSCs was assessed using Cell-counting kit-8 (CCK-8) (BOSTER, Wuhan). After cell counting, BMSCs were seeded onto the studied specimens held by 24-well tissue culture plates at a density of 1 × 10 5 cells/well (six replicates). After incubating for 1 day, 4 days and 7 days, respectively, the culture media were removed and the specimens were rinsed with phosphate buffered saline (PBS) buffered three times to remove the unattached cells. Cell viability was measured by adding 1 mL/well of culture medium containing 100 μL of CCK-8 for 1 h incubation. Then, 110 μL of supernatant from each well was transferred to new 96-well cell culture plates. The optical density value of the supernatant was measured with a microplate reader (Molecular Devices, USA) at 450 nm.
2.2.4
Alkaline phosphatase (ALP) activity assays
After 7 and 14 days of culture with different surface modified composites, alkaline phosphatase (ALP) activity of BMSCs was detected. BMSCs were seeded in 24-well plates at a density of 3 × 10 4 cells/mL (n = 3). After 24 h, the medium was replaced by an osteogenic induction and differentiation complete medium. After 7 and 14 days of culture, 30 mL cell culture medium was taken and the OD value at 520 nm was determined according to the instructions of alkaline phosphatase (ALP) assay kit (Nanjing Jiancheng Bioengineering Institute, Jiangsu, China) based on the conversion of para-nitrophenylphosphate to para-nitrophenol after incubation for 30 min. For normalization, the total protein concentration was measured by a bicinchoninic acid assay protein kit (Biosea Biotechnology Co., Ltd., Beijing). Therefore, ALP was expressed as the total protein content (U/mg protein).
2.2.5
Extracellular matrix (ECM) mineralization assay
After 28 days of incubation, alizarin red staining (ARS) solution (2%) was used to determine the formation of mineralized nodules according to the manufacturer’s instructions. At the required time point, the cells were immobilized with 4% paraformaldehyde for 30 min, then washed with PBS three times. Fixed cells were further washed with distilled water. Then 2% (wt/v) ARS solution was added to stain the newly formed bone-like nodules on the samples with deep red. In quantitative analysis, the stain was dissolved in 10% cetylpyridinum chloride, and the absorbances were measured with a microplate reader at 562 nm (Molecular Devices, USA).
2.3
In vivo osseointegration studies
2.3.1
Animal surgical procedures
Eight-week-old male SD rats (weighing 200–220 g) were selected for in vivo studies. All experimental protocols about the experiments on live vertebrates were approved by Medical Ethics Committee of Shanxi Medical University and conducted in accordance with the ethical principles of Medical Ethics Committee of Shanxi Medical University. Animal surgical procedure was performed under sterile conditions. General anesthesia was administered by intraperitoneal injection. The calvarial defect model was established on the skulls of SD rats with a dental grinder. The diameter of the calvarial defect model was 5 mm and the depth was 1 mm. The sterilized samples were implanted into the skulls. Two parallel samples with the same surface treatment were arranged in two defect models on one rat skull (6 parallel samples were set). Finally, the rats were sacrificed by intravenous injections of air 1, 2 and 3 months post-surgery. Skulls were taken out and washed with water and immersed in formalin solution.
2.3.2
Fluorescent staining
The rats were anesthetized with 10% chloral hydrate. Alizarin Red (30 mg/kg) was injected subcutaneously into the abdomen three weeks before the rats were sacrificed, then calcein (6 mg/kg) was injected subcutaneously into abdomens one week before the rats were sacrificed. After the rats were sacrificed, the hard tissue sections were made and observed by laser confocal microscopy (VK-X200K, KEYENCE, Japan). Meanwhile, mineral deposition rate (MDR) could be obtained by Eq. 1 where D is the average of vertical distance between the two fluorescent marker lines, n = 6; t is the interval time between two fluorescent labeling.
2.3.3
Histological analysis
After the rats were dissected, their skulls were removed, dehydrated and fixed with 4% paraformaldehyde solution for 12 to 24 h. Then, the samples were placed in 10% EDTA for decalcification for about 2 months (EDTA was replaced every two days). The samples after decalcification were dehydrated and embedded with paraffin. The paraffin embedded samples were frozen for 10 min, then sliced with a tissue slicer and baked for 1 h at 60 °C. Then, hematoxylin and eosin (H&E) were used to stain the tissue sections. Finally, the tissue sections stained by H&E were observed with an inverted optical microscope (OPTEC BDS400).
2.3.4
X-ray analysis
After dissection, the complete skulls of the rats with the implanted samples were analyzed by X-ray analyzer (PLX5200, PEALONG).
2.4
Biological safety evaluation
2.4.1
Cytotoxicity test in vitro
Murine fibroblast L929 cells (BOSTER) were procured for cytotoxicity test. Cytotoxicity test on extracts of the samples were carried out according to ISO 10993-5: 2009. The specific method was consistent with our previous work [ ]. In brief, after cultured in the extracts for 1, 4 and 7 days, CCK-8 (BOSTER) solution was added and the OD value was measured by a microplate reader (SPECTRA max 384, China). Specifically, cell culture medium was applied as negative control and the blank control was set up for each plates. The positive control was set up as the complete medium containing 7.5% Dimethyl sulfoxide (DMSO). Each test was carried out in sextuplication. The absorbance of a blank (OD blank ), a negative control (OD negative ) and the samples (OD sample ) were measured. Finally, relative growth rate (RGR) obtained by Eq. 2 was applied to evaluate the cytotoxicity which was determined by the standard in Table 1 [ ]. To analyze the cytotoxicity specifically, an inverted fluorescence microscopy (MF52, Mshot) was used to observe the morphology of the most preventively data of the cells.
RGR (%)=ODsample-ODblankODnegative-ODblank×100%
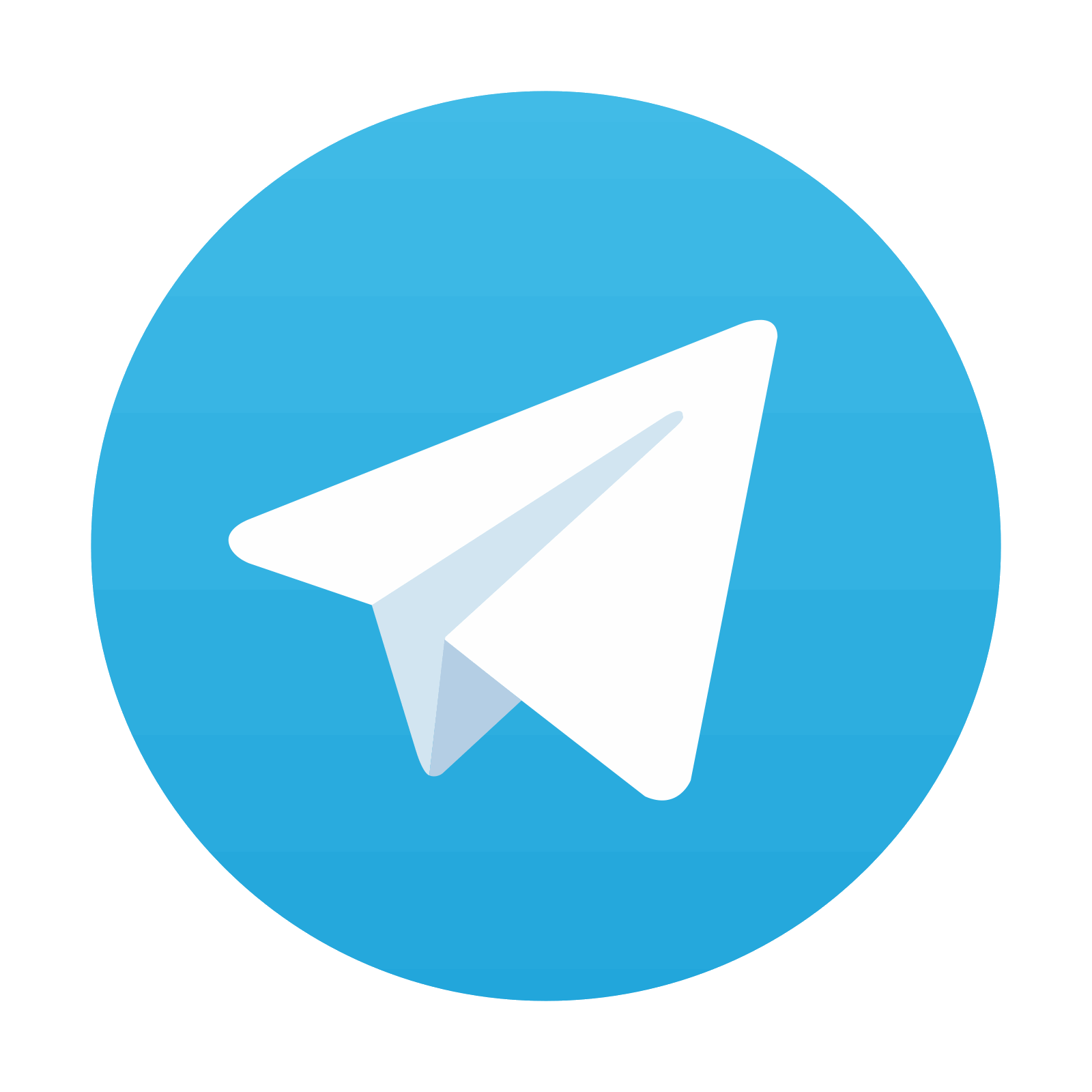
Stay updated, free dental videos. Join our Telegram channel

VIDEdental - Online dental courses
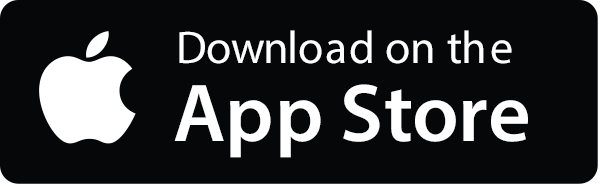
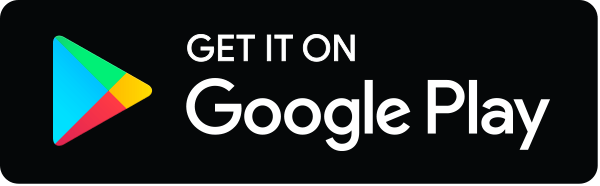
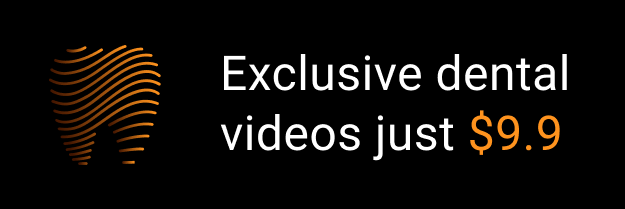