Fig. 9.1
Calcium phosphate precipitation and structures of orthophosphate (Pi) and pyrophosphate (PPi) ions. (a) Calcium phosphate precipitation and solubility at different pH. Left side of equation summarizes that, at pH 6.2, the fraction of monohydrogen and dihydrogen phosphate ions is about equal and that calcium dihydrogen phosphate is 100 times less soluble than calcium monohydrogen phosphate. Right side of the figure shows that phosphate ions exist mostly as monohydrogen phosphate at pH 7. Calcium monohydrogen phosphate precipitates as an amorphous solid that spontaneously rearranges to form hydroxyapatite crystals. (b) Structures of Pi and PPi. Structures are the ionized forms at physiological pH (∼7.2). (Original figures)
9.1.1 Nature of the Apatite Precipitate
The apatite that initially precipitates is crumbly and brittle due to its amorphous (noncrystalline) structure. If the surrounding fluid remains above pH 7, this apatite undergoes a series of spontaneous, solid-state rearrangements whose major end product is hydroxyapatite, a crystal containing ten calcium ions, six phosphate (PO43−) ions, and two hydroxide (OH1−) ions. These changes are due to a spontaneous alkalinization of the apatite, in which monohydrogen phosphate (HPO42−) ions lose a proton and hydroxide ions appear from water molecules trapped in the initial amorphous precipitate (Fig. 9.1a, right). Hydroxyapatite is a long, thin, flat crystal that forms a thick, hard, flat surface that is primarily responsible for the strength of bones and teeth. The long axis is referred to as the “c” axis. The width is the “b” axis and the thickness is the “a” axis.
During bone formation, the crystals form with their “c” axis parallel to the collagen fiber and thicken by accretion at their “a” and “b” axes. During enamel formation, apatite crystals join end-to-end at their “c” axis, forming thin ribbons that become enamel rods.
9.1.2 Apatite Crystal Substitutions Influence Bone Strength and Solubility
The hydroxyapatite crystals in bone and teeth are imperfect due to other anions and cations, especially magnesium, chloride, carbonate, and fluoride ions. Carbonate (CO32−) is the most important. At low carbonate contents (<4% by weight), a carbonate ion replaces a phosphate ion in the crystal (“A” site substitution), but at higher contents (>4% by weight) it replaces a hydroxide ion (“B” site substitution). Either substitution slightly shortens and fattens the crystal (“c” or “a” axes increase) and increases solubility. In contrast, if hydroxide ions are present, they can be replaced by fluoride, which decreases apatite solubility (Sect. 16.2.1). Crystallographic analyses indicate that, in bone and dentin, phosphate is often replaced by carbonate, whereas in enamel it is more often replaced with chloride (Cl1−). Carbonated hydroxyapatite is critical for enamel development (see Sect. 9.5.3).
Enamel mineral has many large hydroxyapatite crystals, whereas bone has many small ones with numerous vacancies and substitutions. These differences increase the elasticity of bone compared with enamel and promote its interactions with the surrounding collagen. Recently, a tightly bound “hydration shell” that fills a porous collagen-apatite junction was discovered around normal bone crystals. The water-filled pores are normally immobile, but repeated stresses cause the water to leak out from between the mineral and collagen. The drying increases mineralization and crystal formation, which may explain the decreased elasticity of bones with age.
9.1.3 Nucleation
Dissolved calcium and phosphate ions may remain soluble despite their concentrations exceeding the solubility product in blood plasma and stromal extracellular (interstitial) fluid where the pH is just above 7 (Sect. 3.3.1). In blood plasma, mineralization is prevented by polyanions, especially albumin, citrate, and pyrophosphate (PPi), which chelate calcium ions and prevent their precipitation with monohydrogen phosphate ions (orthophosphate, Pi, or HPO4 2− ). Pyrophosphate (PPi) inhibits the premature aggregation of calcium with monohydrogen phosphate ions in mineralizing tissues and interstitial fluid throughout the body (Fig. 9.1b).
For mineralization, the normal, metastable state is adjusted by nucleation, measured by the seed and solubility tests. The seed test measures amount of solid apatite required to precipitate Ca2+ and HPO42− ion concentrations exceeding their solubility product. The solubility test measures the minimal concentrations of Ca2+ and HPO42− necessary to induce precipitation. Type I collagen fibers nucleate bone formation as the concentrations of Ca2+ and HPO42− ions increase. Premature nucleation is prevented by pyrophosphate (PPi; Fig. 9.1b), small amounts of which strongly inhibit nucleation.
PPi is made in three ways: (1) in the nucleus as a by-product of RNA synthesis (nNTP → NMPn + nPPi); (2) in the cytosol as a by-product of amino acid activation for protein synthesis (aa + ATP → aaAMP + PPi); and (3) by acetyl CoA synthetase on the outer mitochondrial membrane prior to its degradation for ATP production (R–COOH + ATP + HS–CoA → R–Co–SCoA + AMP + PPi). Amino acid activation is the major source of cytosolic PPi, which is transported by the ANK protein to the osteoid matrix to inhibit premature mineralization (see Sect. 9.3.5).
Summary
Mineralization is the precipitation of calcium and phosphate ions above pH 7. Initial precipitates are soft and noncrystalline (amorphous). If left alone, a solid-state rearrangement slowly and spontaneously forms hydroxyapatite, whose crystals each contain ten calcium (Ca2+), six phosphate (PO43−), and two hydroxide (OH1−) ions. The resulting hard, flat surface is primarily responsible for the strength of bones and teeth. Substituting hydroxide or phosphate ions with carbonate ions increases crystal solubility, whereas substituting hydroxide ions with fluoride ions decreases crystal solubility. In bone, hydroxyapatite crystals have many spaces and substitutions, permitting a water layer between apatite and collagen that dries up and decreases bone’s elasticity with age. Biological fluids are supersaturated with calcium and phosphate, but contain pyrophosphate and polyanions that inhibit spontaneous precipitation. Pyrophosphate interferes with calcium phosphate aggregation and polyanions (citrate, albumin, and other negatively charged proteins) chelate calcium ions and prevent them from being free in solution to precipitate.
9.1.4 The Structures of Bone, Dentin, and Cementum
There are two types of bone tissue: dense (compact or cortical) and spongy (cancellous or trabecular). The difference lies in how tightly the tissue is packed together. Bone matrix is predominantly a mixture of type I collagen fibrils, that resist pulling forces, and calcium phosphate mineral (apatite crystals) that resist compression. The volumes of collagen and mineral in bone are about equal, but the collagen accounts for only ∼20% of the bone weight.
Compact bone consists of closely packed osteons (Haversian systems). In these osteons, central canals called the osteonic (Haversian) canals are surrounded by concentric rings (lamellae) of calcified matrix (Fig. 9.2). Bone cells (osteocytes) lie between the calcified rings, in spaces called lacunae. Small channels (canaliculi containing osteocyte processes) radiate from the lacunae to an osteonic (Haversian) canal to provide passageways for nutrients and excreted products. Each osteonic canal contains a central large blood capillary vessel that parallels the long axis of the bone. The capillaries are connected to each other and to larger blood vessels within a thin fibroblast-rich stroma on the surface of the bone (the periosteum) by mineral-perforating (Volkmann’s) canals.
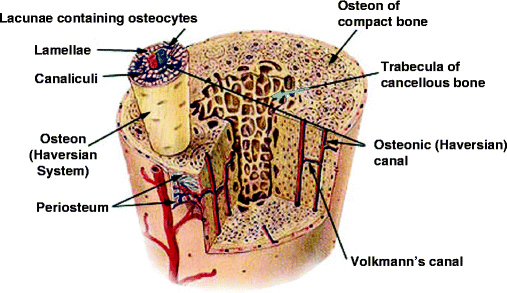
Fig. 9.2
Bone structure. Diagram of a long bone indicating the compact and cancellous structures (see text) (http://training.seer.cancer.gov/module_anatomy/unit3_2_bone_tissue.html; funded by the U.S. National Cancer Institute’s Surveillance, Epidemiology, and End Results (SEER) Program, via contract number N01-CN-67006, with Emory University, Atlanta SEER Cancer Registry, Atlanta, Georgia, U.S.A.)
Cancellous bone is less dense. It consists of thin plates and bars of bone (trabeculae) adjacent to small, irregular cavities (bone marrow) containing a connective tissue from which the various cells that form the red and white blood cells and platelets differentiate (Sect. 11.1.1). The cavities are the equivalent of osteonic canals and are surrounded by trabeculae containing lacunae and canaliculi in which the osteocyte cell bodies and processes are respectively situated (Fig. 9.2). Although the trabeculae appear haphazard, they are in fact organized to provide maximum strength, like braces that support a building. The trabeculae of cancellous bone follow stress lines. Stress creates boney microcracks that activate osteoclasts and osteoblasts (Sect. 10.2.1), leading to appropriate remodeling and realignment. Bone cells develop as osteoblasts in the periosteum or on the surface of trabeculae and become osteocytes in lacunae following matrix mineralization. Osteoblasts and osteocytes account for about 15% of the bone mass.
Dentin is secreted unmineralized like bone. The predentin matrix consists of collagen, glycoproteins and proteoglycans like the osteoid matrix described below (Sect 9.3.1). The collagen fibers aggregate with their long axes parallel to long thin odontoblast processes which extend through the predentin and remain in the mineralized tissue as the center of dentinal tubules. To mineralize the dentin around the tubules, Ca2+ ions are transported to the mineralization front from underlying blood vessels in the developing pulp cavity. The process of mineralization is likely mediated by matrix vesicles as described for bone (Sect. 9.3.1). The innermost lining of dentinal tubules is mineralized last and becomes more dense than the inter-tubular dentin. The dentinal tubules have lateral branches that permit the odontoblasts to communicate with each other like osteocytes. These lateral branches are much more numerous in root dentin than in coronal dentin. Unlike bone, dentin contains no blood vessels. Cementum is deposited in layers above the dentin on the external surface of the root as a calcified matrix for the insertion of Sharpey’s periodontal ligament fibers (Sect. 3.1.5). Cementum is less mineralized than compact bone or dentin.
9.1.5 Two Mechanisms of Mineralization
Intramembranous ossification is responsible for most of the mineralization of the skull, including the maxilla and mandible. It begins with the differentiation and activation of osteoblasts from fibroblast-related precursors within a region of connective tissue that demarcates where the bone will develop. The osteoblasts secrete a nonmineralized protein-rich (osteoid) matrix and, as they move away, the matrix mineralizes (Fig. 9.3a). The periosteum remains uncalcified and contains latent and undifferentiated osteoblasts for bone remodeling. Odontoblasts (Ob) and cementoblasts secrete an osteoid-like matrix similar to that of intramembraneous ossification.
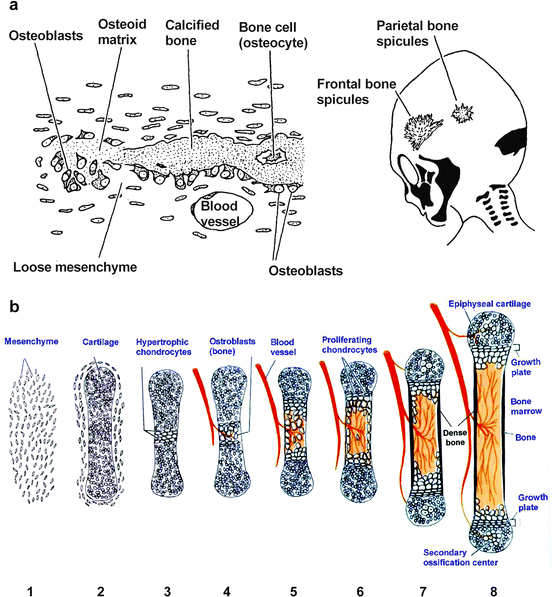
Fig. 9.3
Bone synthesis. (a) Membrane ossification is typified by cranial bone fusion. Osteoblasts differentiate from loose mesenchymal cells resembling primitive fibroblasts. The center of cranial bones becomes cancellous as in long bones. (b) Endochondral ossification is typified by long bone development and growth. In this more complex process, mesenchymal cells aggregate (1) and differentiate into cartilage in the shape of the bone (2). The central region becomes hypertrophic and encapsulated (3). The lack of nutrients causes apoptosis (cell death), which attracts blood vessels and other mesenchymal cells from which osteoblasts differentiate and grow so that bone and periosteum replace the central cartilage (4). As osteoclasts resorb the enlarged central cavity (5), chondroblasts proliferate at each end (6). The periosteum induces appositional growth resembling membrane bone and the central cavity (bone marrow, red) forms cancellous bone, see text (7). At each end of the bone, the chondrocytes become apoptotic, and attract blood vessels so that secondary ossification centers develop above and below the epiphyseal cartilage and bone growth ceases (8) (Adapted from Developmental Biology, Ed. S.F. Gilbert, Sinauer Assoc. Inc., 1997 and copied from Web site http://classes.aces.uiuc.edu/AnSci312/Bone/Bonelect.htm)
Endochondral ossification is responsible for the mineralization of long bones and it begins after chondroblasts have formed a three-dimensional cartilaginous template of the future bone (Fig. 9.3b). Blood vessels grow into the center of the cartilage and osteoblasts develop alongside invading endothelial cells at the growth plate where type II collagen is already present and type X collagen will develop (Sect. 4.3.2). The invading osteoblasts replace the type II and type X collagen of the endochondral growth plate with type I collagen. As the osteoid matrix is laid down, the chondrocytes proliferate and then undergo apoptosis (Fig. 9.3b). Apoptosis is described in Sect. 13.4.1. Traces of type II and type X collagen and proteo-glycosaminoglycans may remain from the cartilage and become ossified. A periosteum forms around the compact outer surface.
Summary
Bone is synthesized by osteoblasts that differentiate from assembled, mesenchymal, fibroblast-like precursors (intramembranous ossification), or from precursors that migrate into cartilage (endochondral ossification). Bone mineralizes over an osteoid matrix composed of type I collagen fibers, which nucleate (initiate) and control the process. Outer surfaces of bone are hard (compact bone) but the insides form a cavity that is poorly mineralized (cancellous bone). The dentin and cementum of teeth resemble compact bone. The outer surfaces of bones are covered by an organic periosteum containing capillaries and an uncalcified cell-rich stroma. The central cavities also contain capillaries and a different fibroblast-like stroma within which blood cells develop. As they form, they enter the circulation where they replenish the red and white cells and platelets that mediate oxygen transport, immunity, and blood clotting.
9.1.6 Secretion of Osteoid Matrix
Skeletal tissue mineralization (bone formation) is initiated by osteoblasts, which secrete the osteoid matrix (Fig. 9.4). They express type I procollagen in secretory vesicles together with matrix vesicles that pinch off from the membrane. The matrix vesicles are pushed away from the cell surface, possibly by the flow of fluid containing calcium and phosphate ions that are also transported through the cell from the extracellular fluid on the outer surface. Collagen fibers develop further away from the cell surface than from fibroblasts.
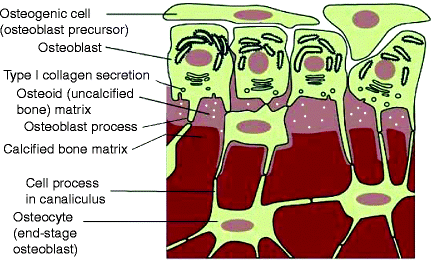
Fig. 9.4
Osteoblast secretion and matrix vesicle formation. The outer surface of all bones is covered by fibroblast-like cells that differentiate into pre-osteoblasts that secrete osteoid matrix to remodel the surface as necessary. The surface osteoblasts extend into the osteoid tissue by long processes that attach to osteocytes (fully differentiated, nondividing osteoblasts) within the bone. Changes in the environment may be sensed by the osteocytes, which transmit them as remodeling signals to the osteoblasts. The osteoid matrix is filled with many small membrane-covered matrix vesicles containing various amounts of precipitated basic calcium phosphate (white circles) (Modified from Fig. 22-52 in The Molecular Biology of the Cell. B. Alberts et al., 4th Ed. 2002. Garland Science, Taylor & Francis Group, New York)
Matrix vesicles are difficult to isolate from developing membrane bone and the only well-characterized matrix vesicles available are from chondroblasts about to be replaced by osteoblasts during endochondral ossification (Fig. 9.3). The chondroblast matrix vesicles shown in Fig. 9.4 are surrounded by cartilage collagens (type II and type X) and aggrecan (Sect. 6.5.1). As osteoblasts invade and secrete their own matrix vesicles and type I collagen, the cartilage collagen and proteoglycans are almost entirely removed, presumably by matrilysins expressed by dying chrondroblasts or invading osteoblasts ahead of type I collagen expression. It is not clear how cartilage derived matrix vesicles shown in Fig. 9.5 are related to osteoblast-derived matrix vesicles but they are assumed to be similar.
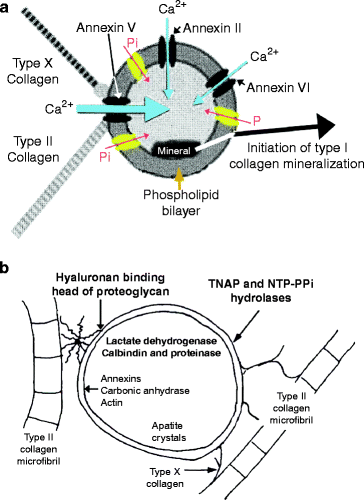
Fig. 9.5
Matrix vesicle composition. (a) Transporters. The major calcium transport channel is made from annexin V (thick blue arrow). Lesser amounts of annexins II and VI are also present (thin blue arrows) but their role in Ca2+ ion transport is uncertain. Calcium ion transport is enhanced by the presence of type II and type X collagen fibers during endochondral ossification. Phosphate transporters (PiT) are shown in yellow with a red arrow indicating the direction of phosphate transport. As each HPO42− ion is transported into the vesicle, a sodium ion is transported out (not shown) (Modified from Fig. 5 of T. Kirsch, Annexins and tissue mineralization: matrix vesicle, ion channel activity of annexins and annexin V/collagen interactions, published in Annexins: Biological importance and annexin-related pathologies (2003). Edited by J. Bandorowicz-Pialuka, Kluwer/Plenum Publishers, 233 Spring St., New York, NY 10013). (b) Matrix vesicle-associated enzymes and proteins. Collagen Type X, the proteoglycan link protein and the hyaluronan binding region of proteoglycans may attach cartilage collagen (type II) to the outer surface of matrix vesicles. Nucleoside triphosphate pyrophosphohydrolase (NTAP) and NTP-PPi hydrolase are anchored in the matrix vesicle membrane. Annexin V and carbonic anhydrase are concentrated just below the matrix vesicle membrane. Lactate dehydrogenase (LDH), calbindin D9K, and proteases are soluble in the center of the matrix vesicle (Modified to refer to osteoblast matrix vesicles from Anderson HC (1992) “Conference introduction and summary.” Bone and Mineral 17:110)
9.1.7 Osteoblast Transport of Calcium and Phosphate Ions to Matrix Vesicles
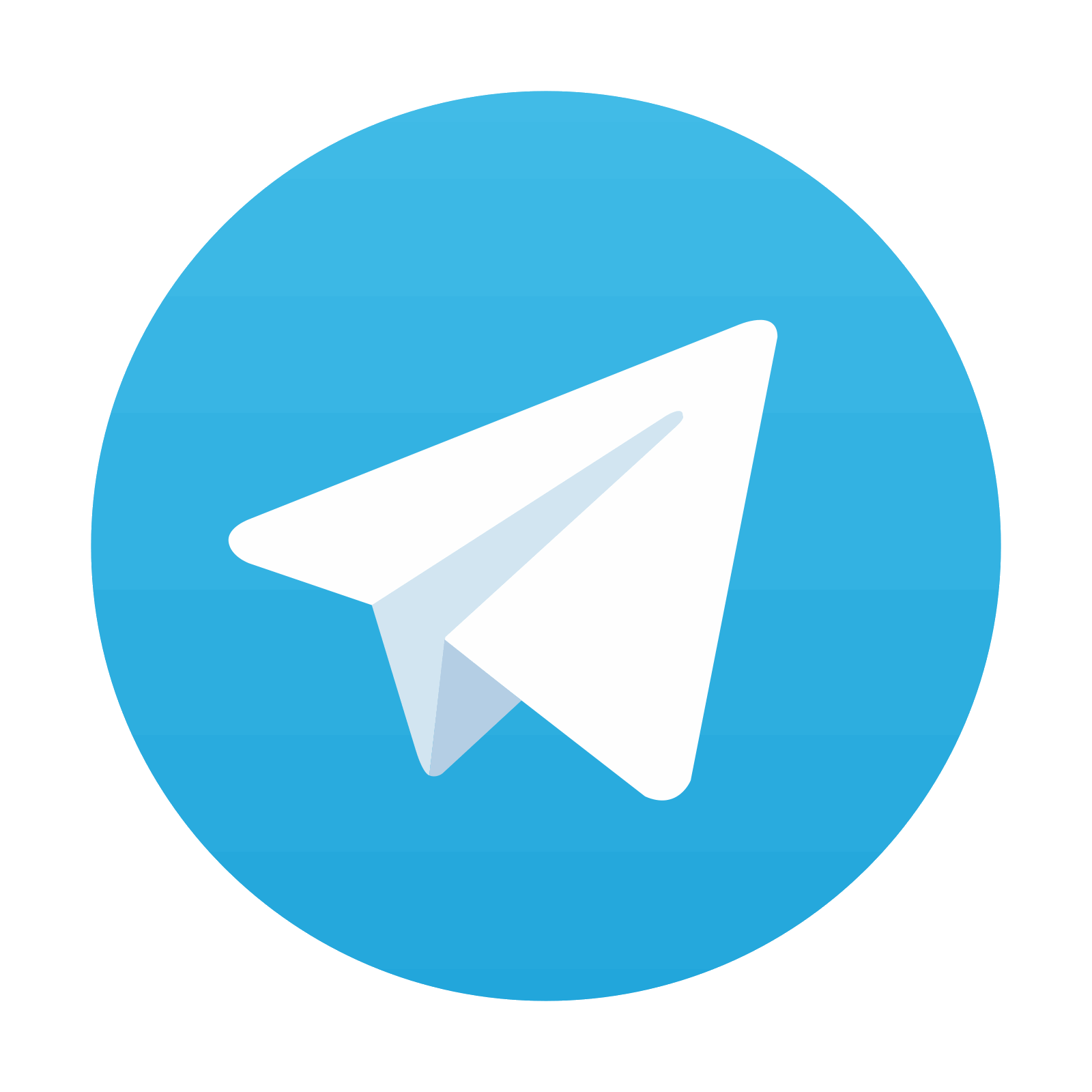
Stay updated, free dental videos. Join our Telegram channel

VIDEdental - Online dental courses
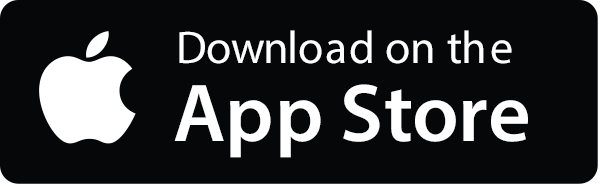
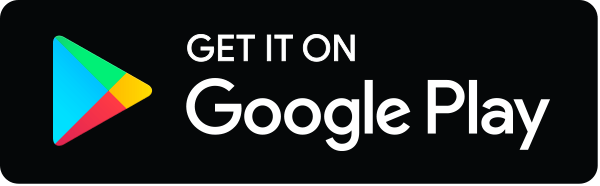