Graphical abstract

Highlights
- •
Oral biofilms are heterogeneous microbial communities critical to health.
- •
Biofilm attributes include constituents (who), quantity, structure, and function.
- •
Measurement methods should be appropriate, and their limitations should be known.
- •
Efforts to improve reproducibility in biofilm experiments are underway.
- •
Normalizing results and using multiple methods reduces results ambiguity.
Abstract
Objective
The goal of this manuscript is to provide an overview of biofilm attributes and measurement approaches in the context of studying biofilms on tooth and dental material surfaces to improve oral health.
Methods
A historical perspective and terminology are presented, followed by a general description of the complexity of oral biofilms. Then, an approach to grouping measurable biofilm properties is presented and considered in relation to biofilm-material interactions and material design strategies to alter biofilms. Finally, the need for measurement assurance in biofilm and biofilm-materials research is discussed.
Results
Biofilms are highly heterogeneous communities that are challenging to quantify. Their characteristics can be broadly categorized into constituents (identity), quantity, structure, and function. These attributes can be measured over time and in response to substrates and external stimuli. Selecting the biofilm attribute(s) of interest and appropriate measurement methods will depend on the application and, in the case of antimicrobial therapies, the strategic approach and expected mechanism of action. To provide measurement assurance, community accepted protocols and guidelines for minimum data and metadata should be established and broadly applied. Consensus standards may help to streamline testing and demonstration of product claims.
Significance
Understanding oral biofilms and their interactions with tooth and dental material surfaces holds great promise for enabling improvements in oral and overall human health. Both substrate and biofilm properties should be considered to develop a more thorough understanding of the system.
1
Introduction
The oral environment represents a seemingly ideal place for microbial growth – warm and moist with abundant nutrients. Yet fluctuations in the oral environment can be harsh. Temperatures can vary from ≈0 °C to ≈70 °C, with large temperature changes sometimes occurring in seconds . The nutrient availability can go from feast to famine. The mechanical forces can be complex and range from resting to high shear or impact associated with drinking, mastication, and oral hygiene. Intake of acidic foods can significantly alter the pH. These excursions from resting conditions in the oral environment can happen within seconds but may take seconds, minutes, or longer to return to resting levels, and recur numerous times over a typical day. It is in this highly dynamic environment that oral microbial communities such as biofilms grow and flourish.
Despite widespread regard of bacteria as harmful, oral biofilms are an integral part of a healthy mouth. They can limit the growth of pathogenic microbial strains, provide environmental stability over time, improve epithelial barrier function, and “educate” the host immune system . They can also serve as a reservoir for fluoride . However, a shift from a healthy state into a pathogenic one, a process termed dysbiosis, can result in tooth decay (caries), gingivitis, periodontitis, and implant infection and failure. Dental caries has remained the number one infectious disease worldwide, with untreated caries in permanent teeth affecting 2.4 billion people in 2010 . Of the estimated 160 million restorations placed in the U.S. in 2005, over half were replacements of failed restorations . Secondary caries has been identified as the primary mode of failure for dental restorative materials, including amalgam, resin-based composite restorations, and glass-ionomer cements . Moreover, oral bacteria are increasingly implicated in other diseases. For instance, DNA from oral bacteria (indicative of the presence of those bacteria) has been found in the extra-oral environment, suggesting possible roles for oral bacteria in systemic health issues ranging from cancer to adverse pregnancy outcomes to cardiovascular disease .
Given the important role of oral biofilms, it is critical to improve our ability to measure and quantify them in order to increase our understanding of their impact on health and disease and our ability to manipulate them, ultimately to improve and maintain oral and overall health. This manuscript is not an exhaustive review of the extensive biofilm literature, but rather a journey into biofilm properties, measurements, and efforts toward measurement assurance, all in the context of oral biofilms relevant to tooth and dental materials. To that end, what do we know and what can we do?
2
Historical perspective and terminology
Living microorganisms in dental plaque were first observed by Antonie van Leewenhoek in 1684 . He described them as “living animalcules” and foreseeably noted, “The number of these animals in the scurf of a mans teeth, are so many that I believe they exceed the number of men in a kingdom .” Other discoveries and findings followed and paved the way toward modern understanding of biofilms. These include the introduction of solid nutrient medium in 1881 by Robert Koch , eventually leading to culturing of microorganisms on agar plates. It was demonstrated in 1940 that the presence of solid surfaces available for bacterial growth increased the amount of bacteria present, especially under reduced nutrient conditions . In 1969, electron microscopy of slime layers from streams revealed the presence of a matrix encasing microbial cells .
While the term “biofilm” may have first been used in publication in 1975 , the ubiquity of biofilms was recognized by Bill Costerton in 1978 . Costerton has been deemed the “founding father of the field of biofilms” for his pioneering work leading to modern biofilm research. Indeed, he defined the term biofilm in 1995 as “matrix-enclosed bacterial populations adherent to each other and/or to surfaces or interfaces” . Over the years, the knowledge on biofilms grew to reflect a deeper appreciation for their complexity and diversity, as did the definition of the term biofilm. In 2002, Costerton further defined a biofilm to be a “microbially derived sessile community characterized by cells that are irreversibly attached to a substratum or interface or to each other, are embedded in a matrix of extracellular polymeric substances that they have produced, and exhibit an altered phenotype with respect to growth rate and gene transcription” .
Biofilms should also be considered in the context of microbiomes. The first use of the term “microbiome” in print is credited to a textbook in 1988, where microbiome was defined as “a characteristic microbial community occupying a reasonably well defined habitat which has distinct physico-chemical properties” . Microbiome was also used by Joshua Lederberg in 2001 “to signify the ecological community of commensal, symbiotic, and pathogenic microorganisms” . Biofilms can therefore be considered a subset of microbiomes, in the sense that each biofilm is an ecological community. Of note, microbial communities in the oral environment can be biofilms ( e.g. , dental plaque) or non-biofilms ( e.g. , salivary microbiome).
Overall, oral biofilms play an important role in microbiology, from the microorganisms that van Leeuwenhoek visualized in 1684 to the microbiome studies of today, likely due to the importance of oral microbes and their ease of accessibility.
2
Historical perspective and terminology
Living microorganisms in dental plaque were first observed by Antonie van Leewenhoek in 1684 . He described them as “living animalcules” and foreseeably noted, “The number of these animals in the scurf of a mans teeth, are so many that I believe they exceed the number of men in a kingdom .” Other discoveries and findings followed and paved the way toward modern understanding of biofilms. These include the introduction of solid nutrient medium in 1881 by Robert Koch , eventually leading to culturing of microorganisms on agar plates. It was demonstrated in 1940 that the presence of solid surfaces available for bacterial growth increased the amount of bacteria present, especially under reduced nutrient conditions . In 1969, electron microscopy of slime layers from streams revealed the presence of a matrix encasing microbial cells .
While the term “biofilm” may have first been used in publication in 1975 , the ubiquity of biofilms was recognized by Bill Costerton in 1978 . Costerton has been deemed the “founding father of the field of biofilms” for his pioneering work leading to modern biofilm research. Indeed, he defined the term biofilm in 1995 as “matrix-enclosed bacterial populations adherent to each other and/or to surfaces or interfaces” . Over the years, the knowledge on biofilms grew to reflect a deeper appreciation for their complexity and diversity, as did the definition of the term biofilm. In 2002, Costerton further defined a biofilm to be a “microbially derived sessile community characterized by cells that are irreversibly attached to a substratum or interface or to each other, are embedded in a matrix of extracellular polymeric substances that they have produced, and exhibit an altered phenotype with respect to growth rate and gene transcription” .
Biofilms should also be considered in the context of microbiomes. The first use of the term “microbiome” in print is credited to a textbook in 1988, where microbiome was defined as “a characteristic microbial community occupying a reasonably well defined habitat which has distinct physico-chemical properties” . Microbiome was also used by Joshua Lederberg in 2001 “to signify the ecological community of commensal, symbiotic, and pathogenic microorganisms” . Biofilms can therefore be considered a subset of microbiomes, in the sense that each biofilm is an ecological community. Of note, microbial communities in the oral environment can be biofilms ( e.g. , dental plaque) or non-biofilms ( e.g. , salivary microbiome).
Overall, oral biofilms play an important role in microbiology, from the microorganisms that van Leeuwenhoek visualized in 1684 to the microbiome studies of today, likely due to the importance of oral microbes and their ease of accessibility.
3
Biofilm features
The current understanding is that a biofilm is the preferred way of life for many microorganisms, as community lifestyle provides resilience and adaptability to favor survival. Oral biofilms could arguably be some of the more resilient communities, given the range of conditions they must endure daily. Bacterial growth into three-dimensional, matrix encased biofilm communities leads to emergent properties, including genotypic and phenotypic spatial heterogeneities as well as increased antimicrobial resistance, as discussed herein.
The biofilm life cycle ( Fig. 1 ) can be described as a multi-stage process . The initial step is cell attachment to a surface. In the oral cavity, the surfaces of teeth and dental restorations are not pristine – they are covered with a salivary pellicle upon exposure to saliva. This conditioning film results from adsorption of salivary components, such as proteins, carbohydrates, and lipids, to biotic and abiotic surfaces in the oral cavity . Initial bacterial attachment is therefore mediated by pellicle components. The presence of a pellicle changes the surface chemistry and can affect bacterial adhesion, such as adhesion of oral streptococci to orthodontic brackets . Initial adhesion is reversible and mediated by long range interactions such as van der Waals forces and electrostatic interactions. It transitions to irreversible adhesion via mechanisms such as specific binding of adhesins to the surface . Adherent cells then proliferate into microcolonies and eventually form a mature biofilm. After maturation or in stressful conditions, a biofilm can undergo dispersal via organized release of cells from the community, which can enable colonization of new sites . This process of biofilm formation requires time and resources ( e.g. , nutrients).
The matrix surrounding the cells is termed the extracellular polymeric substance (EPS) and represents “biopolymers of microbial origin in which biofilm microorganisms are embedded” . Initially thought to be simply exopolysaccharides, the EPS is currently recognized as a highly hydrated structure that includes polysaccharides, proteins (including enzymes), glycoproteins, glycolipids, lipids, and extracellular DNA (e-DNA) . These non-cellular components of biofilms are thought to have a protective role: providing support structures to shield microbial cells in biofilms from shear forces and host cells, serving as nutrient reservoirs, reducing diffusion, and maintaining cell–cell proximity to promote interactions. Moreover, the matrix can be indicative of biofilm virulence .
The three-dimensional community lifestyle of biofilms results in internal chemical gradients that contribute to spatial heterogeneities in structure and function throughout the biofilm. Unlike planktonic cultures in laboratories where the majority of the cells are thought to have a similar phenotype ( e.g. , dividing in the presence of nutrients, stationary phase when nutrients are depleted), cells within biofilms are genotypically and phenotypically heterogeneous. Water channels often penetrate biofilm structures to allow oxygen and nutrients to better infiltrate the biofilm. Cells closest to the channels may experience a very different local environment as compared to cells deeper within the microcolonies . As an example of genotypic heterogeneities, many oral bacteria are facultative or strict anaerobes, yet they thrive in the oral environment. Their ability to survive is attributed to oxygen gradients that can create anoxic environments within the biofilm , as well as cellular responses that protect against oxidative stress . Likewise, pH gradients can also develop , leading to spatial variations in structure and function.
The phenotypic heterogeneities in biofilms include cell metabolic activity. The microbial continuum of life goes from alive and actively dividing to dead, with many intermediate points . Defining and measuring the viability and activity of an individual cell (or an overall biofilm) is difficult, particularly due to the changes in viability and activity that can depend upon factors such as location within the biofilm and nutrient conditions . Dormant cell states such as persister cells and viable but not culturable (VBNC) cells are part of this continuum, but their place on the continuum is unclear. While it has recently been proposed that persister cells might represent a transition state between culturable and VBNC cells , the relationships among the various states are poorly understood and difficult to measure .
Persister cells are genetically unchanged cells with extremely low metabolic activity and can be found in both planktonic and biofilm cultures . Their low activity level makes them tolerant to the many antimicrobials that affect metabolically active cells, e.g. , via targeting translation or transcription. After the environmental stress has subsided, persister cells can return to a metabolically active state and regrow the biofilm. Due to this behavior, persister cells have been likened to cancer stem cells, which are also resistant to treatments and can enable a tumor to re-emerge . It is thought that a small fraction of microbial cells stochastically transition to a persister state . In addition, environmental conditions have been shown to induce persistence by triggering a stress response such as shifts in toxin-antitoxin systems or upregulating DNA repair genes after DNA damage due to antibiotics . The study of persister cells is ongoing within the biofilm community and includes efforts to elucidate mechanisms of their formation as well as develop approaches to increase their susceptibility to antimicrobials.
Persister cells are not the only aspect of biofilms that contribute to antimicrobial resistance and tolerance . It is likely a combination of factors that enable biofilms to result in recalcitrant infections that require up to 1000-fold higher concentrations of antimicrobials as compared to planktonic cells . For instance, biofilm characteristics including reduced diffusivity due to matrix components, antimicrobial sequestering by matrix components, cell–cell interactions such as horizontal gene transfer, and cell metabolic state can contribute to this response . In the oral cavity, relevant measurements of biofilms on tooth and dental materials can lead to improved understanding of the role of biofilm attributes in the resultant biofilm function, including antimicrobial resistance.
4
Biofilm properties
The general attributes of biofilms can be divided into four main categories: constituents, quantity, structure, and function ( Fig. 2 ). These attributes can be measured over time to characterize biofilm formation and response to perturbations. Table 1 lists example properties for each category and example techniques that could be used to measure those attributes. This list is designed to provide a context for the discussion on biofilm measurements and is not exhaustive. Each technique listed, as well as those not listed, has its own assumptions and limitations that should be considered during selection of a technique and interpretation of results.
Category | Example property | Example measurement methods a |
---|---|---|
Constituents (identity) | Phenotype(s) | Gram staining, morphology, biochemical methods, growth conditions, serotyping |
Genotype(s) | qPCR, 16S rRNA and whole genome sequencing, metagenomics, mass spectrometry | |
Matrix composition | Biochemical assays, fluorescent dyes, microscopy, FTIR and NMR spectroscopy | |
Quantity | Biomass | Crystal violet, dry mass, optical density |
Total cells | Total DNA, qPCR, dPCR, microscopy, agar growth, MPN, flow cytometry | |
Differential cell count | qPCR, dPCR, selective agar, microscopy, flow cytometry | |
Matrix | Biochemical assays, stains, FTIR spectroscopy | |
Structure | Spatial organization of cells | Optical microscopy, SEM, fluorescent staining |
Spatial organization of matrix | SEM, optical microscopy | |
Mechanical properties | Rheology, AFM | |
Function | Viability/vitality | CFUs, plating efficiency, modified qPCR, microscopy, membrane integrity, ATP activity, gene expression |
Metabolic activity | Tetrazolium salts, microscopy, biochemical assays | |
Acid production | pH, chemical assays, indirect methods such as demineralization and degradation measurements | |
Chemical signaling | Metabolomics, biochemical assays | |
Gene expression | Transcriptomics, RT-PCR, proteomics | |
Adhesion forces | AFM, induced shear assays |
a Abbreviations: AFM – atomic force microscopy; ATP – adenosine triphosphate; CFU – colony forming unit; dPCR – digital PCR; FTIR – Fourier transform infrared; MPN – most probably number; NMR – nuclear magnetic resonance; PCR – polymerase chain reaction; qPCR – quantitative PCR; rRNA – ribosomal ribonucleic acid; RT-PCR – reverse transcription-PCR; SEM – scanning electron microscopy.
4.1
Constituents
The constituents of a biofilm are the materials that make up the biofilm, including the cellular (bacteria, fungi, etc.) and non-cellular (viral particles, extracellular matrix, debris, small molecules, etc.) components.
Traditional methods to characterize cellular constituents rely on phenotypic identification primarily via culture-based and biochemical methods . Culture-based techniques include the use of selective agar and determination of appropriate culture conditions (temperature, pH, etc.) and nutrient requirements. Biochemical methods often focus on metabolic pathways and enzymatic activity. Other techniques such as Gram staining and morphology of cells and colonies are also applied. These methods typically require a pure culture of the strain of interest, which may be challenging for polymicrobial biofilms, and make assumptions that the strain will fit into known patterns.
The advent of genomics-based approaches has greatly advanced the identification of microorganisms, particularly for those not yet culturable in vitro . The increased application of next generation sequencing based on whole genome sequencing shotgun approaches or 16S rRNA targeted approaches has led to a greatly increased appreciation for the microbial diversity in the oral environment. Genomic studies of oral microbial communities suggest that over 600 different species are present in the oral microbiome , with the composition varying with factors such as age, location in the mouth, and time of day . This diversity in composition confirms that each community responds and adjusts to its specific niche. For instance, biofilm cellular constituents have been shown to vary based upon restoration material. One study demonstrated that biofilms on glass-ionomer cements have lower genotypic diversity as compared to those on resin composite and amalgam . While we do not fully understand the mechanisms and implications of these differences, it does leave open the possibility (or promise) for new materials designed to foster a particular biofilm composition with reduced pathogenicity.
Other emerging approaches to identify microorganisms include proteomics-based methods and matrix assisted laser desorption ionization time of flight mass spectrometry (MALDI-TOF MS) , recently reviewed by Singhal et al. . Mass spectrometry techniques have been shown to differentiate strains of mutans streptococci to the subspecies level . Approaches such as these add to the toolkit for microbial identification to enhance overall capabilities and provide orthogonal techniques for increased confidence in results.
Identification of matrix components can be indicative of the microbial species present and their activity and function. Methods such as NMR spectroscopy and Raman microscopy have been applied to define the chemical composition of the matrix. Understanding matrix composition may provide information on potential targets for biofilm disruption or removal. For instance, methods to purify and characterize polysaccharides within a biofilm may help to identify polysaccharides specific to acidogenic biofilms that could serve as targets for biofilm dispersal therapies. As with many aspects of biology, redundancy is built into the matrix, and a multi-pronged approach may be needed to fully disrupt the biofilm.
4.2
Quantity
Quantity refers to the amount of material. Herein, quantity is focused on the amount (mass, number, concentration, etc.) of the constituents present.
Overall biomass measurements reflect the total amount of biofilm material and could be used to evaluate biofilm dispersal or removal technologies ( e.g. , to measure how much material remains). Biomass can also be used to normalize other measurements, such as viability or specific functional measures, to aid in analysis of results. Biomass methods typically measure all cells regardless of viability state, debris from cells and the environment, and matrix components, and therefore cannot accurately reflect cell number, viability, or activity. (See Section 5.4 for more information.)
Quantity can also represent the total number of cells present. Cell counting can be performed using direct or indirect methods. Direct counts physically enumerate the cells in a given area or volume ( e.g. , by microscopy) and extrapolate to the entire sample. Indirect counts look at an aggregate measurement of a cellular component such as total DNA quantity via fluorescent dyes. In addition to total counts, differential counts can be applied to distinguish populations of cells, such as by phenotype or genotype. Differential counts can be absolute or relative ratios of the cell types present and can help indicate shifts in the microbial composition. In many instances, a direct count of viable cells is used to represent the quantity of cells in a given sample due to the challenges in counting all bacteria and the desire to focus on viable bacteria for some applications. Viable cell count is discussed in more detail in Section 4.4 .
The matrix represents the majority of the biofilm dry mass, estimated to be as much as 90% of the dry mass in some biofilms . Given the role of the matrix in caries formation , quantification of total EPS or its individual components can help characterize a biofilm. EPS can be evaluated in situ , such as via fluorescent staining, microscopy, and image analysis . EPS can also be extracted from the biofilm and quantified via biochemical assays and other techniques. For instance, total protein, lipids, or carbohydrates can be measured, as can specific glycosyl linkages .
4.3
Structure
Besides characterizing the identity and quantity of biofilm constituents, it is important to consider how the constituents are arranged , as the structural organization of constituents within multispecies biofilm can directly impact function and response to perturbation. Biofilm architecture can also affect the types of organisms present, as the non-random spatial arrangement of cells defines cell–cell proximity and neighboring cell types, leading to physical and chemical cell–cell interactions such as horizontal gene transfer, metabolic cooperation/competition, and quorum sensing. For instance, the obligate anaerobe Fusobacterium nucleatum coaggregates with oxygen tolerant species in oral biofilms in order to serve as a bridge that allows other obligate anaerobes, which do not coaggregate well with oxygen tolerant species, to join the biofilm . Even within monospecies biofilms, characterization of biofilm structure can shed light on biofilm properties . For multispecies systems, oral biofilms are among the most studied in terms of spatiotemporal organization . Oral bacteria found in dental plaque (oral biofilms) have been classified into early and late colonizers based on studies using enamel surfaces in the oral cavity (with saliva). Systematic studies on the effects of restorative material properties on the sequence of cell adhesion and the overall biofilm architecture could elucidate driving forces that lead to differences in the resulting biofilms. Based on this knowledge, materials could possibly be designed to alter biofilm development.
Structure can be measured directly, e.g. , via microscopy to map cells and extracellular components. It can also be measured indirectly by methods that quantify bulk material properties influenced by the architecture of the biofilm. For instance, biofilms can be considered as viscoelastic materials, where the matrix is akin to a cross-linked polymer gel. Resulting mechanical properties including elastic, storage, and loss moduli; yield stress; and tensile strength can shed light on overall biofilm structure.
4.4
Function
Biofilm function, such as viability, metabolic activity, pathogenicity, gene expression, or adhesion, is of primary interest for most applications. For oral biofilms, an often studied function is acidogenicity as it can lead to caries formation. Other functional measures could be indicative of a biofilm that resists pathogens or provides other benefits. Function can be affected by the identity, quantity, and structure of the constituents as well as the surrounding environmental conditions, and can in turn affect these other attributes.
Viability is typically used to reflect a cell’s ability to grow. Viability can be presented as a ratio of viable to total cells ( e.g. , percent viability) when the total cell count is known. In situations where the total cell count is uncharacterized, an aggregate viability measurement that considers relative differences among samples can be used. The most common measurement of viability is based on colony forming units (CFUs) determined by inoculating a dilution series of the cells onto nutrient-rich agar . CFU, with all its flaws, remains the most direct measurement for a cell’s ability to grow. This method has its own assumptions and limitations and is most appropriate for situations where dead cells and cell debris are not of interest. Data from CFU measurements can be normalized to total cell count to obtain the plating efficiency ( e.g. , CFU/mL divided by the total cells/mL). Plating efficiency reveals more about the state of the biofilm, but can be a challenging measurement for biofilms, particularly because uniform and complete biofilm dispersal can be difficult to achieve (see Section 5.3 ).
Indirect measures of viability rely on various cell properties that may be altered with viability state. These measures are sometimes referred to as vitality measurements as they represent a specific function of a vital cell but do not directly indicate the ability to grow. One such set of measurements relies upon cell membrane integrity (see Section 5.2 for limitations with depending on membrane integrity alone). A number of commercially available fluorescent dyes and “live/dead” assays claim to differentiate viable and dead cells based on membrane integrity . In other approaches, modified qPCR protocols utilize an intercalating molecule such as propidium monoazide (PMA) to enter cells with compromised membranes and bind their DNA to prevent amplification . Only DNA from cells with intact membranes should then be available for amplification and detection. Other viability measurements focus on measuring ATP or RNA . Methods should not and do not always agree with one another , as they measure different properties of the biofilm. With a number of options available, the method selection process should consider the expected mechanism of cell death, if possible. For instance, bacteriostatic effects of antimicrobials that alter intracellular machinery ( e.g. , antibiotics that disrupt protein synthesis) might not be detectable with assays that detect membrane integrity.
Metabolic activity is another key function in biofilms. Activity can be represented a number of ways, such as via a snapshot of the bulk metabolic function at a given time , characterization of metabolism in individual cells using microscopy , or evaluation of gene expression or metabolic byproducts. Bulk metabolic activity is related, but not identical to, viability and vitality, as biofilms often have viable but metabolically inactive cells such as dormant and persister cells. As metabolic activity is dependent on nutrient availability, cells should have appropriate nutrients to stimulate the desired activity on a measurable level. Adding fresh nutrients to “wake-up” cells may be particularly important for static cultures where nutrients may have been consumed during biofilm growth , as seen with 24 h Streptococcus mutans biofilms that have a large biomass but little metabolic activity .
One pathogenic function relevant to oral biofilms is cariogenicity, which can be characterized via measurements such as acid production, matrix production, or demineralization potential. Numerous biofilm compositions can give rise to this same pathogenic function. For instance, S. mutans is implicated in caries formation , yet not all carious biofilms contain S. mutans . Thus if the desired outcome is reduced cariogenic activity, a functional measure of acid production or local pH may be sufficient, and a characterization of the type or amount of constituents may not be necessary.
Specific activity within a biofilm may also be measured by gene expression via targeted and untargeted approaches. Targeted approaches can include qRT-PCR and arrays. For instance, a multi-species transcriptome array was developed and used to evaluate the effect of Streptococcus mitis on gene expression in S. mutans biofilms . Untargeted approaches such as metatranscriptomics evaluate the entire transcriptome of a microbial community. They have been used to study expression of virulence factors in oral biofilms related to the progression of periodontitis and differences in gene expression between patients with and without periodontitis . RNA sequencing (RNA-Seq) has also been used to detect gene expression patterns related to active caries . The emerging field of metabolomics can help profile small molecules such as metabolic byproducts and cell signaling molecules that are critical to biofilm function .
Adhesion is a function critical for biofilm formation and survival. Adhesive forces can represent the interactions between a bacterium and another bacterium or a surface, such as between oral bacteria and enamel . The number of cells that adhere to a surface, for instance after washing with buffer or applying a known shear force, can indirectly represent the adhesion of a particular cell–substrate interaction . Cohesive forces within biofilms are important for maintaining the structural integrity of the biofilm and are relevant for biofilm dispersal technologies. Cohesiveness has been evaluated using techniques such as AFM or rheology .
4.5
Biofilm–substrate interactions
Properties of a biofilm contribute to its response to and effects on the environment, including the substrate. Insights into interactions between biofilms and tooth or dental materials in the host environment have emerged in recent years, bringing recognition to the highly dynamic nature of these interactions ( Fig. 3 ). The properties of the restorative material can influence the biofilm , and the biofilms can alter the materials. For instance, cariogenic biofilms can promote surface degradation of resin-modified glass ionomers , and esterases from oral bacteria and saliva can degrade resin-based composite materials . Leaching of these degradation products as well as unincorporated monomers and photoinitiators can in turn alter bacterial growth and gene expression . Although the focus herein is biofilm measurements, it is equally important to characterize the substrate to have a more complete picture of the system and the biofilm–substrate interactions.
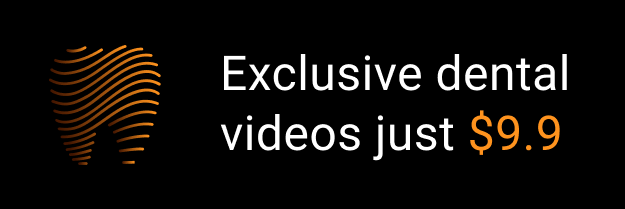