1
Bioceramics in Dentistry
Vineeta Nikhil, Sachin Gupta, Shikha Jaiswal, and Padmanabh Jha
Department of Conservative Dentistry and Endodontics, Subharti Dental College, Meerut, UP, India
1.1 Introduction
Biomaterials as described by the American National Institute of Health are natural or synthetic substance(s) other than drugs that can be used for therapeutic or diagnostic medical purposes to maintain or improve the quality of life[1]. Along with biocompatibility, biological sustainability is also a very important property of any biomaterials that are intended to be used to reconstruct body function for an unspecified duration. However, materials are also required for temporary support of functions. Therefore, depending on the tissues to be replaced and function required, different types of materials are used as a biomaterial, e.g. metal, ceramic, polymer, hydrogel, or composite.
Ceramics are inorganic, non‐metallic materials that are hard, brittle, heat‐resistant, and corrosion‐resistant. In addition to their biocompatibility, ceramics can be obtained with biostable, bioactive, or bioresorbable properties making them eligible to be used as biomaterials. Ceramic base biomaterials that are specially developed for biological applications (both medical and dental) are categorized as Bioceramics. Porous bioceramics (BC) can facilitate neo‐angiogenesis and neo‐osteogenesis inside their porous structure. Additionally, resorbable bioceramics get replaced by the newly formed desired tissues.
Josette Camilleri described bioceramics in endodontics as the materials that are composed of tricalcium silicate‐based cement synthesized from lab‐grade chemicals and that do not include aluminum in their composition [2].
1.2 History and Evolution of Bioceramics
- Portland cement that was obtained from the limestones coming from Portland got patented in 1824 [3, 4].
- According to Peltier, the use of plaster of Paris, a resorbable ceramic, was first described by Dressman in 1892 to fill the bone cavities which were later found to be filled with solid bone [5].
- In the early 1920s, the use of calcium phosphates as a stimulus to osteogenesis for bone defect repair started [6].
- Use of ceramic hydroxyapatite (HA) granules for bone defect repair was first reported in the early 1950s [7].
- In 1963, Smith worked on a ceramic bone substitute, Cerosium [8].
- In 1969, researchers found a new material called bioglass that could be easily integrated into human bone [9].
- In the 1980s, the first hydroxyapatite coated implants were marketed.
- LeGeros et al. in 1982 used calcium phosphate in restorative dental cement as a bioceramics material [10].
- In 1984, the use of bioceramics started as a root canal sealer [11].
- The first self‐hardening calcium phosphate cements (CPCs) were developed in 1986 [12].
- MTA was developed in the Loma Linda University, California, and was first documented in 1993 [13, 14] as a retrograde filling and perforations repair material.
- Chevalier et al. in 1997 found that the friction between zirconia and alumina is very low.
- In 1998, “TH‐Zirconia” implants were introduced.
- The United States witnessed the first commercial MTA product, ProRoot MTA (Dentsply Tulsa Dental Specialties, Johnson City, TN) in 1999.
- In 2009, Septodont, France, marketed the calcium silicate‐based product “Biodentine” as a permanent bulk dentin substitute.
- Angelus was the first company to launch a paste/paste bioceramic root canal sealer (MTA‐Fillapex) in 2010.
- In 2019, Bio‐C® Temp, a ready‐to‐use bioceramic paste for intracanal dressing was developed by Angelus, Brazil.
Earlier in the 1950s, bioceramics were used in dentistry because of their inertness and good biocompatibility that had no reaction with living tissues, e.g. zirconia, alumina, and carbon. They were primarily used for the fabrication of dental implants and prosthesis. Later on, the development of bioactive ceramics, e.g. bioglass (45S5) by Hench [9], extended the scope of these bioceramic materials as they offered in vivo benefits by inducing biomineralization (i.e. formation of apatite crystal layer). Bioglass (45S5) is composed of 45% SiO2, 24.5% CaO, and 24.5% NaO2. The addition of 6% P2O5 by weight enhanced the bioactivity of the glass [15]. However, this glass material was very weak and brittle. In the 1980s, the trend changed toward using implant ceramics that react with the environment and produce newly formed bone.
This bioglass was later modified to create variants by adding magnesium, borates, etc., for improving mechanical and setting properties of bioceramics [3].
Acknowledging the bioactivity property of bioceramics and their application into dentistry as mineralizing and regenerative materials has brought enormous productive changes [16].
Bioactive materials such as sintered hydroxyapatite (HA) [17] and β‐wollastonite (CaO–SiO2) in an MgO–CaO–SiO2 glass‐based matrix [18, 19] have been developed for over the last four decades [20].
Ternary CaO–MgO–SiO2 system‐based glass ceramics possessed better mechanical and chemical properties and thus are suitable materials for wear resistance, biomedical, and ceramic coating applications [21–23]. The addition of fluorides of Ca and Mg to substitute Na2O in the conventional composition (SiO2–CaO–Na2O–P2O5) led to the development of antibacterials and bioceramics with higher flexure strength and hardness [20]. Ion substitutions (Ca2+, Mg2+, and B3+) decreased the coefficient of thermal expansion of the bioactive glass ceramics [19]. In addition to bioglass, calcium silicate and aluminate based bioceramics also showed the property of biomineralization.
Although the shift from older to newer formulations is quite slow, many bioceramic materials have been developed that overcome the previous drawbacks.
1.3 Classification of Bioceramics
Bioceramics are classified on the basis of their generations, interaction with tissues, structure, composition, resorbability, and uses.
1.3.1 Based on Generations
Bioceramics are divided into three generations:
- First generation: The first generation bioceramics are inert, thus do not initiate any reaction with living tissues, e.g. zirconia and alumina. Although they are biocompatible, for the body tissue they are like a foreign body, leading to the formation of an acellular collagen capsule which isolates them from the body tissues.
- Second generation: In the 1980s, the trend changed toward the development of bioceramics with improved bioactivity and the second generation bioactive bioceramics were developed, e.g. calcium phosphates, glasses and ceramic glasses, and calcium silicate. These bioceramics can react with the physiological fluids forming biological‐type apatite as a byproduct of said reaction; in the presence of living cells, this apatite can form new bone.
- Third generation: The third generation bioactive, porous bioceramics were developed because of biological requirements. Only porous ceramics can fulfil physiological requirements in their use as scaffolds for cells and inducting molecules and being able to drive self‐regeneration of tissues. Example: nanometric apatites, shaped in the form of pieces with interconnected and hierarchical porosity, within the micron range so that cells can perform their bone formation and regeneration tasks.
1.3.2 Based on Tissue Interaction
The fact that the reactivity of solids begins on their surface is of particular importance in the field of bioceramics because on application they remain in contact with an aqueous medium and in the presence of cells and proteins [24]. Based on different types of interactions [25, 26] shown by bioceramics, it is classified as:
- Bioinert: These materials have a high chemical stability in vivo; thus, they do not interact and show no chemical changes when they are in contact with living tissues. They also possess high mechanical strength, e.g. alumina, zirconia, and carbon.
- Bioactive: Bioactive bioceramics have the character of osteoconduction and the capability of chemical bonding with living bone tissue. These materials bond directly with living tissues by undergoing interfacial interactions, e.g. bioactive glasses, HA, calcium silicates, and calcium aluminates.
- Biodegradable: These materials when in contact with living tissues either become soluble or resorb and eventually get replaced or incorporated into tissue, e.g. tricalcium phosphate, calcium phosphate, aluminum–calcium–phosphates, and calcium aluminates.
1.3.3 Based on Structure
Depending on the structure type, bioceramics are classified into:
- Dense: Bioceramics that are available as solid bulk structures like bars, rods, or to any shape through injection molding fall in this category. Because of their nonporous nature, these bioceramics show poor vascularization and osteoinduction ability, e.g. zirconia.
- Porous: Porous bioceramics have attracted tremendous attention with their excellent biological function and osteoinduction ability. They provide scaffolds for cells to adhere, proliferate, differentiate, and regenerate tissues. The mean size and surface area of porosity plays an important role in the growth and migration of a tissue into the bioceramic scaffolds, e.g. CaP scaffold.
1.3.4 Based on Composition
On the basis of their composition, bioceramics are classified into:
- Calcium silicate‐based: The calcium silicate‐based bioceramics can be further categorized on the basis of their application:
- Cement: e.g. Biodentine (Septodont, France), mineral trioxide aggregate (MTA), Portland cement.
- Sealer: BioRoot RCS (Septodont, France), Endo‐CPM‐Sealer (EGO SRL, Buenos Aires, Argentina), MTA Fillapex (Angelus, Brazil), TECHBiosealer (Profident, Kielce, Poland).
- Calcium phosphate‐based bioceramics: These materials are obtainable as bone cements, paste, scaffolds, and coatings. The tricalcium phosphate has shown the property of osteogenesis during bony defect treatment, e.g. tricalcium phosphate and HA. Bioglass, a glass ceramic containing calcium and phosphate, showed bonding with the living bone with a calcium phosphate‐rich layer [27].
- Mixture of calcium silicates and calcium phosphates: EndoSequence BC Sealer (Brasseler, Savannah, GA, USA)/Total Fill, BioAggregate (Innovative Bioceramix Inc., Vancouver, Canada), Tech Biosealer, Ceramicrete (developed at Argonne National Lab, IL, USA), iRoot BP, iRoot BP plus, iRoot SP (Innovative Bioceramix Inc., Vancouver, Canada)
- Calcium aluminate‐based: These materials can set, harden, and maintain their physical and mechanical properties over time in an oral environment. They have the ability to create apatite on their surface and provide tight seal between the tooth and itself. The cements can also contribute to the healing of the dental pulp or in the tissue surrounding the root of a tooth by eluting ions to stimulate cytokines, e.g. EndoBinder, Generex, Capasio, and Quick‐set.
1.3.5 Based on Resorbability
- Nonresorbable: Alumina, zirconia, carbon, HA, and calcium phosphate cement.
- Resorbable: β tricalcium phosphate and calcium sulfate
1.3.6 Based on Their Location‐Specific Use in Endodontology
They are classified as [28]:
- Intracoronal
- Pulp capping materials
- Regenerative endodontic cements
- Intraradicular root canal sealers
- Apical plug cements
- Perforation repair cements
- Extraradicular
- Root‐end filling materials
- Perforation repair cements
1.4 Forms of Bioceramics
Bioceramics are available in different forms and phases:
- Powder or microspheres
- As a thin coating on a metal or polymer
- Porous 3D structure
- Composites with a polymer component
- Solid dense structure.
1.4.1 Alumina
Aluminum oxide (Al2O3) is commonly known as alumina. It is highly inert and resistant to corrosion even in a highly dynamic oral environment. Additionally, it has high wear resistance and surface finish. As an implant material, it was first used in the 1970s. It does not integrate with bone or soft tissues. Because of its hardness being higher than the other metal alloys, alumina found its main application as biomaterials in the articular surfaces of joint replacements [29].
1.4.2 Zirconia
Zirconium dioxide is commonly known as zirconia. As zirconium is a very strong metal, it is also known as “ceramic steel.” The inherent properties of zirconia such as inertness, high toughness, strength, wear resistance, fatigue resistance, and biocompatibility make it suitable to be used as dental bioceramics. Zirconia established itself as implant material in the 1960s. Compared to zirconia, partially stabilized zirconia showed superior flexural strength, fracture toughness, lower stiffness, and a superior surface [30].
1.4.3 Hydroxyapatite (HA)
HA (Ca10(PO4)6(OH)2) is a major component of human bones and teeth. It belongs to the calcium phosphate family with a calcium to phosphorus ratio of 1.67. Since most of the inorganic portion of the human bone tissue is HA, it can be effective in reconstructing human bone tissue. It is capable of integrating and supporting bone growth, without breaking down or dissolving. It has higher stability in aqueous media than other calcium phosphate ceramics within a pH range of 4.2–8.0 [31]. In the 1970s, resorbed residual ridge repair started with HA and in 1988 in North America it was declared as a successful implant material.
To fill the bone defects or spaces, HA may be used in the form of either powder, blocks, or beads. The bone filler acts as a scaffold to facilitate the formation of natural bone.
HA is also used to alter the surface properties of metals by the application of coating on its surface. Because of the poor mechanical properties, HA cannot be used for load‐bearing applications.
1.4.4 Calcium Phosphate
Examples of calcium phosphate‐based bioceramics used in dentistry are CPC, tricalcium phosphate, HA, and bioglass that are used as bone substitutes and also an adjunct with the dental cements [25]. CPC offers the potential for in situ molding and injectability.
Tricalcium phosphate is a biodegradable bioceramic. Tricalcium phosphate has four polymorphs; the most common ones are the α and β forms. It dissolves in physiological media and can be replaced with bone during implantation.
When the ratio of Ca/P in calcium phosphate compounds is less than 1, it becomes highly soluble and is thus unsuitable for biological implantation. It is used as a coating on metallic implants, as fillers in polymer matrices, as self‐setting bone cements, as granules or as larger shaped structures.
1.4.5 Mineral Trioxide Aggregate
Dr. Torabinajed introduced MTA in 1993. MTA powder comprises 75% mixture of tricalcium silicate (CaO)3SiO2, dicalcium silicate (CaO)2SiO2, and tricalcium aluminate (CaO)3 Al2O3 20% bismuth oxide; and 5% gypsum. This combination possesses osteoconductive, osteoinductive, and biocompatible properties. When mineral trioxide powder is mixed with water, initially calcium hydroxide and calcium silicate hydrate are formed [32]. MTA is an active biomaterial with the potential to interact with the fluids in the tissues. The pH value is 10.2 after mixing and it rises to 12.5 at three hours resulting in an alkaline environment [32].
1.4.6 Biodentine
Biodentine, a tricalcium silicate‐based hydraulic cement, was developed by Septodont research group (Septodont, Saint‐Maur‐des‐Fosses, France) as a bioactive dentin substitute material. Tricalcium silicate is the main component and the additives include calcium carbonate in the powder; calcium chloride, water‐soluble polymer, and water make the liquid. Calcium chloride controls the setting time. Biodentine exhibits a higher initial rate of calcium ion release compared to other similar material types [33, 34]. This is due to the interaction of the calcium carbonate that enhances the reaction rate [35].
Hydrated calcium silicate gel and calcium hydroxide are produced because of the hydration of tricalcium silicate. The hydrated calcium silicate gel and calcium hydroxide gradually fill in the spaces between the tricalcium grains by precipitating at the surface of the particles. Biodentine continues to improve in terms of internal structure toward a denser material, with a decrease in porosity after the initial setting.
1.5 Physicochemical Properties of Bioceramics
The physiochemical properties of bioceramics govern the application and outcome of the use of bioceramic materials. The therapeutic effect of these biomaterials in aiding healing and restoring function is dependent on the chemical reactions that affect their setting, hardening in the presence of oral tissues and fluids. The biological response of the tissues is mediated through a dynamic interaction between these materials, depending on their composition, biocompatibility, and specific properties such as surface microhardness, flow, pH, flexural strength, etc. related to them. The following discussion is focused on the physiochemical properties of commercially used bioceramics commonly used in endodontics.
1.5.1 Portland Cement
Portland cement (PC) offers antibacterial activity, biocompatibility, bio‐inductivity, and acceptable physical and chemical properties when used for varied applications in dentistry, particularly endodontics. The physical and chemical properties of Portland cement resemble more closely to MTA.
- PC like MTA is available as gray and white.
- Discoloration – Ordinary PC (gray) shows lesser discoloration compared to gray MTA. However, there is an equal lack of discoloration seen by white MTA and white PC [36].
- Solubility – Greater solubility is seen with MTA when compared to white PC. It also shows better washout resistance compared to MTA in different solutions [36].
- Bioactivity – Maturation of MTA after hydration is more structured than PC, hence the former displays better bioactivity. Calcium ion release and formation of HA crystals is seen with both gray and white PC [36].
- Particle size – The particle size of white ProRoot MTA is significantly smaller than white PC both before and after hydration [36].
- Antibacterial properties – PC shows antibacterial and antifungal properties similar to MTA against Enterococcus faecalis, Micrococcus luteus, Staphylococcus aureus, Staphylococcus epidermidis, Pseudomonas aeruginosa, and Candida albicans [ [36]].
- Sealing ability – White and gray MTA had similar sealing abilities as a root‐end filling material when checked by means of dye penetration as compared to white and gray PC. However, when checked as a perforation repair material by means of protein leakage, white PC showed better sealing ability compared to white and gray MTA [36].
- Biocompatibility – Cell culture studies have shown variable result per the cell type. Essentially, there has been no genotoxicity or cytotoxicity seen associated with PC similar to MTA with respect to fibroblasts. However, with respect to human bone marrow derived mesenchymal stem cells, MTA displayed greater proliferation and migration compared to PC. Biomineralization is greater with MTA compared to PC when observed at 30 and 60 days. Pulpotomy performed with PC and MTA is successful both clinically and radiographically, but the root canals showed greater obliteration with PC [36].
1.5.2 ProRoot MTA
ProRoot MTA is made of fine hydrophilic particles that set in the presence of water. It seals off pathways between the root canal system and surrounding tissues, significantly reducing bacterial migration. Its excellent compatibility with the dentinal wall allows for a predictable clinical healing response. The physical and chemical properties of ProRoot MTA are:
- pH value: The pH value of MTA is 10.2 after mixing and rises to 12.5 after three hours. White MTA (WMTA) displays a significantly higher pH value 60 minutes after mixing compared to Gray MTA (GMTA) [37].
- Compressive strength: The compressive strength of ProRoot MTA is 40 MPa at 24 hours and ~67 MPa at 21 days [36].
- Setting time: The recommended powder liquid ratio for MTA is 3 : 1. The setting time of gray ProRoot MTA has been reported by Torabinejad et al. as 2 hours and 45 minutes (±5 minutes). The mean setting time of MTA has been reported to be approximately 165 minutes, which is longer than the amalgams, Super EBA and IRM. GMTA has significantly higher initial and final setting times than WMTA. Islam et al. reported final setting times of 140 minutes (2 hours and 20 minutes) for WMTA and 175 minutes (2 hours and 55 minutes) for GMTA. The presence of gypsum is reported to be the reason for the extended setting time. pH‐hydrated MTA products have an initial pH of 10.2, which rises to 12.5 three hours after mixing.
- Pushout bond strength: The retentive strength of MTA is significantly less than that of glass ionomer or zinc phosphate cement and, thus, it is not considered to be a suitable luting agent. Studies have shown that a 4‐mm thickness of MTA (apical barrier) offered more resistance to displacement than a 1‐mm thickness. One of the study found the push‐out bond strength of MTA after 24 hours to be ~5.2 ± 0.4 MPa. The strength significantly increased to 9.0 ± 0.9 MPa after the samples were allowed to set for seven days [36].
- Flexural strength: Raghavendra et al. in their review reported that placement of moist cotton pellets over the setting MTA for 24 hours showed significant increase in flexural strength, i.e. ~14.27 ± 1.96 MPa [36].
- Porosity: The amount of porosity in mixed cement is related to the amount of water added to make a paste, entrapment of air bubbles during the mixing procedure, or the environmental acidic pH value [36].
- Microhardness: Less humidity, low pH values, the presence of a chelating agent, and more condensation pressure might adversely affect MTA microhardness [36].
- Sealing ability: The majority of the dye and fluid filtration studies suggest that MTA materials overall allow less microleakage than traditional materials when used as an apical restoration while providing equivalent protection as a zinc oxide eugenol (ZOE) preparation when used to repair furcation perforations. GMTA and WMTA are shown to provide equivocal results compared against gutta‐percha when used as a root canal obturation material in microleakage studies. No significant leakage is observed when at least 3 mm of MTA remains after root‐end resection. However, significantly more leakage is seen when 2 mm or less thickness of MTA remains after root‐end resection [36].
- Particle size: The physical properties of cement might be influenced by crystal size. Smaller sized particles increase surface contact with the liquid and lead to greater early strength and ease of handling [36].
1.5.3 MTA Angelus
MTA Angelus exhibits a reduced setting time, is sold in containers that permit more controlled dispensing, and possesses the same desirable properties as traditional MTA.
- Setting time: The setting time of MTA Angelus is approximately 14 minutes, which is considerably less than WMTA and GMTA [38].
- pH value: The results on the pH and calcium ion release of MTA Angelus are conflicting. While one of the studies suggests that MTA Angelus produced a higher pH value and calcium ion release than GMTA within 168 hours after mixing while other reported that pH and calcium release is lower in MTA Angelus than in MTA. Yet another study concluded that the pH and calcium ion release between MTA and MTA Angelus is not significantly different [38].
- Microhardness: The microhardness of MTA Angelus has been reported to be increasing with incubation time and influenced by the technique of mixing [38].
- Sealing ability: Several dye leakage studies have compared the quality of the seal by MTA Angelus, zinc‐free amalgam, Vitremer (a resin‐modified glass ionomer cement), and Super EBA, with conflicting reports. Wang in a review reported that MTA Angelus gave the best seal against root dentin among all the tested materials. In contrast, another study found more leakage with MTA Angelus and Vitremer compared to Super EBA in apical sections. However, no significant difference could be found between MTA Angelus and Super EBA in other tooth sections. Controversy also exists between MTA Angelus and MTA. One study showed no significant difference in dye penetration between them, whereas GMTA showed less dye leakage when used as a perforation repair material in another investigation. When an internal matrix was used for MTA Angelus, it demonstrated a better seal [38].
- Radiopacity: MTA Angelus has also shown to have a lower radiopacity than WMTA and GMTA [38].
1.5.4 Biodentine
”Biodentine” is a calcium silicate‐based product that became commercially available in 2009. The material is formulated using the MTA‐based cement technology and possesses better physical and biological properties compared to other tricalcium silicate cements such as mineral trioxide aggregate (MTA) and BioAggregate.
- Setting time: The setting time of Biodentine according to manufacturer’s instructions is 9–12 minutes. The presence of setting accelerator in Biodentine results in faster setting, thereby improving its strength and handling characteristics [36]. Grech et al. compared the setting times of Biodentine, zirconium replaced tricalcium silicate cement, and BioAggregate and concluded that Biodentine had the shortest setting time among tricalcium silicate cements (ProRoot MTA, MTA Angelus, etc.).
- Density and porosity: A study done by De Souza et al. compared Biodentine to other silicate‐based cements, IRoot BP Plus, Ceramicrete, and ProRoot MTA using micro‐CT characterization. No significant difference in porosity has been found between IRoot BP Plus, Ceramicrete, and Biodentine [39].
- Compressive strength: During the setting of Biodentine, the compressive strength of Biodentine increases up to 100 MPa in the first hour and 200 MPa at the 24th hour. It continues to improve with time over several days reaching 300 MPa after one month, which is comparable to the compressive strength of natural dentin, i.e. 297 MPa [36]. Biodentine had the highest compressive strength in the study when compared to other tested materials because of the low water/cement ratio used [40].
- Flexural strength: Flexural strength of Biodentine recorded after two hours has been found to be 34 MPa [36].
- Microhardness: In the study done by Grech et al. Biodentine showed superior value of microhardness when compared to BioAggregate and IRM. Goldberg et al. found the microhardness of Biodentine to be 51 Vickers Hardness Number (VHN) at two hours and 69 VHN after one month [40].
- Radiopacity: ISO 6876:2001 has established that 3 mm Al is the minimum radiopacity value for endodontic cements. Grech et al. studied the radiopacity of tricalcium silicate cement, BioAggregate, and Biodentine and concluded that all the materials had radiopacity values greater than 3 mm Al [40].
- Microleakage: Biodentine is found to be associated with high pH 12 and releases calcium and silicon ions that stimulate mineralization. This creates a mineral infiltration zone along the dentin‐cement interface that imparts a better seal [40].
- Marginal adaptation and sealing ability: Micromechanical adhesion of Biodentine allowed excellent adaptability of Biodentine crystals to the underlying dentin. According to a study, MTA and IRM were significantly superior to Biodentine in terms of marginal adaptation when used as a root‐end filling material [40].
- Bond strength: Hashem et al. concluded that Biodentine has low strength during the initial stages of setting, hence the application of a final overlying resin composite restoration (laminated or layered) should be delayed for more than two weeks to achieve adequate bond strength of matured Biodentine to withstand contraction forces caused by polymerization shrinkage of resin composite [40].
1.5.5 BioAggregate
BioAggregate™ is a novel material introduced for use as a root‐end filling material. It is tricalcium silicate‐based, free of aluminum, and uses tantalum oxide as radiopacifier. BioAggregate contains additives to enhance the material performance.
- Properties of BioAggregate
Tuna et al. assessed the long‐term fracture resistance of human immature permanent teeth filled with BioAggregate, MTA, and calcium hydroxide. They suggested that BioAggregate‐filled immature teeth demonstrate higher fracture resistance than the other groups at one year. Considering the long‐term risk of cervical root fracture associated with immature teeth, the use of BioAggregate as a root canal filling material appears to be the most advantageous of the materials tested [41].
Saghiri et al. investigated the compressive strength of MTA, a nanomodification of white MTA and BioAggregate after its exposure to a range of environmental pH conditions during hydration. The authors concluded that the force needed for the displacement of the nanomodification of white MTA was significantly higher than for Angelus White MTA and BioAggregate. They stated that the more acidic the environmental pH, the lower is the compressive strength [42].
Hashem and Amin compared the effect of acidic environment on the dislodgement resistance of MTA and BioAggregate when used as perforation repair materials. They concluded that MTA is more influenced by acidic pH than BioAggregate [43].
- Sealing Ability and Success
El Sayed and Saeed evaluated and compared the sealing ability of BioAggregate versus amalgam, IRM, and MTA. They reported that BioAggregate has a high sealing ability. The authors considered utilizing BioAggregate as an alternative to MTA [44].
1.5.6 Ceramicrete
Ceramicrete‐D is a self‐setting material composed of HA powder, phosphosilicate ceramic, and cerium oxide radiopaque filler, although it may also contain bismuth oxide as a radiopacifier. The pH of the material is reported differently in two separate studies. Porter et al. in their review mentioned different pH values of Ceramicrete reported by different authors range from alkaline to acidic pH [45].
The radiopacity of Ceramicrete‐D is similar to that of root dentin and fulfills the requirement of ISO 6876/2001, although it is lower than that of white ProRoot MTA [46].
The material’s handling and washout resistance properties are superior to those of white ProRoot MTA. Its setting time is 150 minutes. Ceramicrete‐D’s compressive strength is significantly lower than that of white ProRoot MTA [46].
It has been claimed that the material has the potential for bioactivity in the presence of phosphate‐containing fluids. It also has significantly better sealing ability compared to white ProRoot MTA [46].
1.5.7 Calcium‐Enriched Mixture Cement
Calcium‐enriched mixture (CEM) cement is a powder/liquid material.
Raghavendra et al. in their review reported the introduction of a new endodontic material by Asgary et al in 2008 to combine the superior biocompatibility of MTA with appropriate setting time (less than one hour), handling characteristics, chemical properties, and reasonable price. This newly formulated biomaterial, calcium‐enriched mixture (CEM) cement, has been made using different calcium compounds [36].
1.5.7.1 Physical Properties
pH: CEM cement and white ProRoot MTA have no significant difference in pH (10.61 versus 10.71), working times (4.5 minutes versus 5 minutes), or dimensional changes (0.075 versus 0.085 mm). However, there have been significant differences between the materials’ setting times, film thickness, and flow [46].
CEM cement produces an alkaline pH and releases calcium in a similar manner to white ProRoot MTA. In addition, CEM cement releases significantly higher levels of phosphate compared to PC and white ProRoot MTA during the first hour after mixing.
Radioopacity: CEM cement radiopacity is reported to be 2.227 mm Al, which is lower than that of ProRoot MTA (5.009 mm Al) and MTA – A (4.72 mm Al). CEM cement’s radiopacity did not fulfill the requirement of ANSI/ADA specification numbers 57/2000 and ISO 6876/2001 each for endodontic sealing materials (3 mm Al) [46].
Particle size: The particle size of CEM cement is between 0.5 and 30 μm. The percentage of the particle size between 0.5 and 2.5 μm diameter in CEM cement is significantly higher than that in white ProRoot MTA and white PC [46].
The effect of using CH, ProRoot MTA, and CEM cement on flexural strength of bovine root dentin after 30 days showed that all tested materials significantly decreased flexural strength compared to the control [46].
Push‐out bond strength of CEM cement as root‐end filling material is comparable with white ProRoot MTA. Both materials showed higher resistance to displacement when the root‐end preparation has been performed with ultrasonic technique rather than Er, Cr: YSGG laser [46].
1.5.7.2 Antibacterial Activity
Two separate antibacterial investigations evaluated the activity of CEM cement, gray and white ProRoot MTA, PC, and CH on E. faecalis, P. aeruginosa, Escherichia coli, and S. aureus
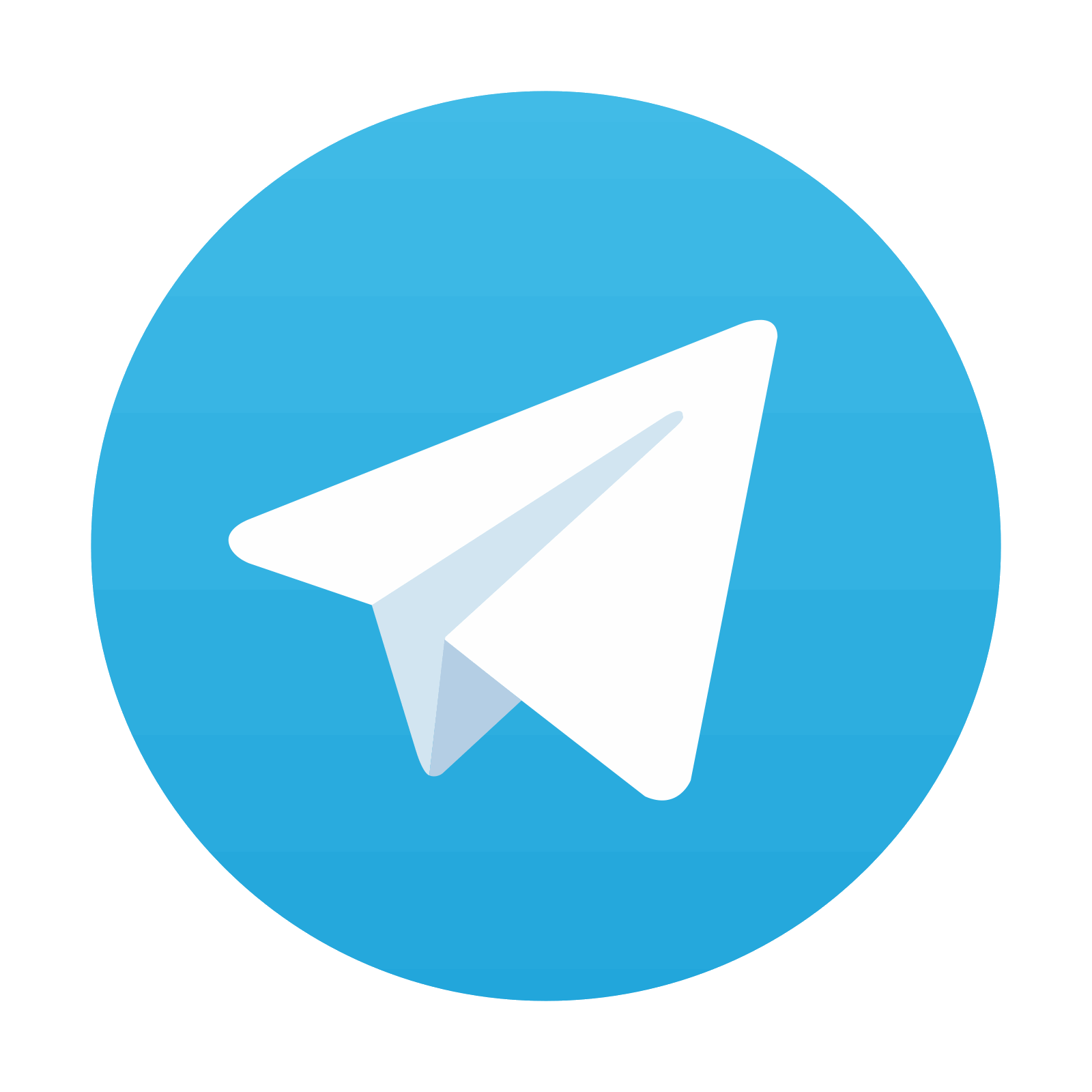
Stay updated, free dental videos. Join our Telegram channel

VIDEdental - Online dental courses
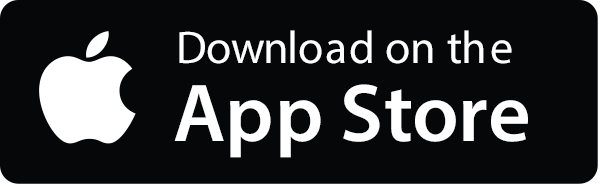
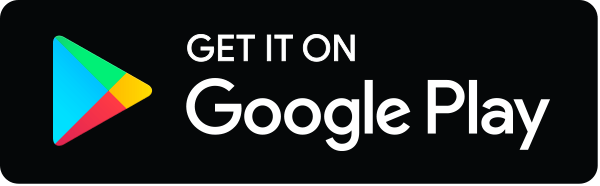