Abstract
Objective
This study systematically reviews the literature on self-healing microcapsule technology and evaluates the biocompatibility of self-healing microcapsules and the efficiency of crack repair within resin-based dental composites.
Methods
An electronic search was carried out using the following databases: MedLine ( PubMed) , Embase, the Cochrane Library and Google Scholar . All titles and abstracts of the articles and patents found were analysed and selected according to the eligibility criteria. Only studies published in English were included; the outcomes sought for this review were dental resin composites with self-healing potential. There were no restrictions on the type of self-healing system involved in dental resin composites.
Results
The search yielded 10 studies and 2 patents involving self-healing approaches to dental resin composites. According to the current literature on self-healing dental resin composites, when a crack or damage occurs to the composite, microcapsules rupture, releasing the healing agent to repair the crack with a self-healing performance ranging from 25% to 80% of the virgin fracture toughness.
Significance
Self-healing strategies used with resin composite materials have, to date, been bioinspired. So far, self-healing microcapsule systems within dental composites include poly urea-formaldehyde (PUF) or silica microcapsules. The main healing agents used in PUF microcapsules are DCPD monomer and TEGDMA-DHEPT, with other agents also explored. Silica microcapsules use water/polyacid as a healing agent. All self-healing systems have shown promising results for self-repair and crack inhibition, suggesting a prolonged life of dental composite restorations. More investigations and mechanical enhancements should be directed toward self-healing technologies in dental resin composites.
1
Introduction
Composites in dentistry are widely used as they have the ability to bond to the tooth structure, repair damaged or decayed teeth to an acceptable aesthetic standard; and have satisfactory mechanical properties and ease of use as a direct-filling material [ ] or as an indirect restoration. Although they are the most common restorative material of choice in today’s dentistry [ , ], composite restorations have been shown to encounter two main downsides: secondary caries and bulk fracture [ ]. The longevity and durability of resin composites are still limited and one of the primary reasons for failure is fracture, with half of all restorations failing in less than 10 years [ ].
The survival rate of composite restorations can be affected by many factors such as patient compliance (caries risk), operator performance and material properties [ , ]. Composite restorations often fail due to the accumulation of micro-cracks resulting from factors such as masticatory forces and thermal stresses [ ]. Development of composite materials aims to increase fracture resistance and improve the service life of dental restorations [ ]. Composites are still brittle and prone to fracture in large cavities with high stress bearing areas [ ], hence the need to inhibit fracture and crack propagation in resin-based restorations is considered fundamental [ ]. The healing potential and repair strategies found in living organisms has inspired material designers to include self-healing mechanisms to increase longevity of materials [ ]. However, these bioinspired approaches do not necessarily involve mimicry of natural biological processes which are too complex to replicate [ , ].
Microencapsulation is defined as “a technology of packaging solids, liquids or gaseous materials in miniature, sealed capsules that can release their contents at controlled rates under the influence of specific conditions” [ ]. Typically, microencapsulation facilitates the delivery of reactive components in various applications ranging from cosmetics to advanced coatings and nutrient retention [ ]. Promising outcomes in minimalizing enamel demineralisation have been shown with remineralizing orthodontic cements and pits and fissure sealants incorporating polyurethane microcapsules with different bioactive water mixtures such as Ca(CO 3 ), NaF and/or K 2 HPO 4 for fluoride release [ ].
The potential for repairing crack damage and mechanical performance recovery in a polymeric resin matrix occurs when mechanical damage allows active materials to be released from the microcapsule and repair the damage [ , ]. Self-healing mechanisms have been achieved in bulk thermosetting polymers [ , ], fibre reinforced composites [ ], dental resin composites [ ], adhesives [ ], elastomers [ , ], and coatings [ ]. Also, nanocapsules enabling healing of submicron crack propagation have been accomplished [ ], i.e. self-healing bonding resins [ ].
The purpose of this review is to gather and reflect upon the ongoing progression in autonomic self-healing microcapsules with dental resin composites ( Fig. 1 ), and to take the opportunity to categorise different types of microcapsule healing systems as described in the literature.

2
Methods
2.1
Electronic databases
The systematic review was conducted according to Preferred Reporting Items for Systematic Reviews and Meta-Analysis (PRISMA) guidelines [ ]; only systematic qualitative synthesis was implemented at the exclusion of quantitative synthesis (meta-analysis). The literature search was carried out by two independent reviewers (KA and NS). An electronic search from 1998 to 2018 was performed using the following databases: MedLine ( PubMed) , Embase, the Cochrane Library and Google Scholar. The search strategy involved the following keywords: (Self-healing OR Self-sealing OR Microcapsules) AND Resin composites, (Self-healing OR Self-sealing OR Microcapsules) AND Dental composites.
2.2
Screening and study selection
All titles and abstracts of the articles and patents found were independently analysed and selected by two reviewers according to the eligibility criteria ( Fig. 2 ). Only studies published in English were included; the outcomes sought for this review were dental resin composites with self-healing potential. There were no restrictions on the type of self-healing system involved in dental resin composites. Hand-searching of reference lists by the articles was carried out to identify missing literature. After duplicate removal, a full text assessment was undertaken against the inclusion and exclusion criteria.

3
Results
3.1
Study selection process
The initial search retrieved 128 articles. After removing duplicates, 127 studies were screened on the basis of title and abstract. 116 articles were excluded as they did not satisfy the selection criteria, leaving 11 articles for full text assessment against the eligibility criteria. One Chinese-language paper was excluded (non-English literature). 10 articles were included for qualitative synthesis, but no articles were included for quantitative synthesis (meta-analysis). The patent search revealed two patents for self-healing microcapsules with dental composites. A summary of the selection process ( Fig. 3 ).

3.2
Study characteristics
Table 1 summarises the included articles about self-healing dental composites. Table 2 summarises the patents. All articles and patents were published between 2010 and 2018. The majority of self-healing systems in dental composites used a microcapsule healing approach; the differences were in the shell materials and the healing agents involved. Poly urea-formaldehyde (PUF) capsular shells were the most common, followed by silica microcapsules. In PUF microcapsules, the healing agents used were DCPD monomer, TEGDMA-DHEPT, with other agents also explored. The healing agent used in silica microcapsules was water/polyacid. Other reinforcing inorganic fillers varied among the selected studies.
Authors | Self-healing system | Self-healing concept & repair mechanism |
---|---|---|
Wertzberger et al. [ ] | PUF microcapsules | Bioinspired SHDC, using (DCPD monomer) microcapsules and Grubb’s catalyst. Some experimental models required external intervention to initiate healing. Average healing performance 57% recovery rate. |
Then et al. [ ] | Melamine-modified UF microcapsules | Bioinspired SHDC, using melamine UF microcapsules (DCPD monomer) with no catalyst in the composite model. No healing capability was studied, only mechanical properties and microcapsules performance evaluated. |
Wu et al. [ ], Wu et al. [ ], Wu et al. [ ] | PUF microcapsules | Bioinspired SHDC, using (TEGDMA-DHEPT amine) microcapsules and benzoyl peroxide (BPO) catalyst in the composite mixture. Average healing performance 65–81% recovery rate. They developed a triple action dental composite with antibacterial, remineralizable and self-healing potential. |
Huyang et al., 2016 [ ] | Silanized silica microcapsules | Bioinspired SHDC, glass ionomer cement (GIC) repair technology made of contemporary dental materials plus silica microcapsules (aqueous solution of polyacrylic acid) and a healing powder (strontium fluoroaluminosilicate particles) in the composite mixture. Average healing performance up to 25% recovery rate. |
Sharma et al. [ ] | Silanized silica microcapsules | Bioinspired SHDC, GIC repair technology made of contemporary dental materials plus silica microcapsules (aqueous solution of polyacrylic acid) and a healing powder (strontium fluoroaluminosilicate particles) in the composite mixture. No healing capability was studied, only static and dynamic mechanical responses evaluated. |
Kafagy [ ] | PUF microcapsules | Bioinspired SHDC, using microcapsules of TMPET, UDMA, and Bis-GMA or a mixture of these monomers as healing agents and (MBDMA) amine. A catalyst mixture consisted of benzoyl peroxide (BPO) and phenyl acetate solvent (PA) in another microcapsules. Average healing performance around 40% recovery rate. |
Chen et al. [ ] | PUF microcapsules | Bioinspired SHDC, using (TEGDMA-DHEPT amine) microcapsules and benzoyl peroxide (BPO) catalyst in the composite mixture. They reported the formulation design and synthesis of a protein-resistant dental composite composed of 2-methacryloyloxyethyl phosphorylcholine (MPC) that also can self-repair damage. Average healing performance 57–71% recovery rate. |
Yahyazadehfar et al. [ ] | Silanized silica microcapsules | Bioinspired SHDC, GIC repair technology made of contemporary dental materials plus silica microcapsules (aqueous solution of polyacrylic acid) and a healing powder (strontium fluoroaluminosilicate particles) in the composite mixture. Testing durability in terms of SHDC resistance to fracture and healing capacity of damage under monotonic and cyclic loading. |
Patent | Inventor | Country | Title | Year | Self-healing system | Claims | |
---|---|---|---|---|---|---|---|
US9763858B2 | Gross and Latta [ ] | United States | Self-healing dental restorative formulations and related methods | 2014 | Polyoxymethyleneurea (PMU) microcapsules, DCPD healing agent | Self-healing dental composites | |
US9931281B2 | Sun [ ] | United States | Multi-functional self-healing dental composites, methods of synthesis and methods of use | 2018 | Silanized silica microcapsules, GIC repair technology | Self-healing, antibacterial, reminerizable dental composites |
4
Discussion
4.1
Self-healing strategies
Self-healing approaches are either bioinspired or biomimetic mechanisms. Nature’s ability to self-heal has inspired engineers and chemists who aim to restore the mechanical properties of a material by suggesting different healing approaches [ ]. The bioinspired approach is considered achievable following observation of healing in natural systems, e.g. coagulation mechanism [ , ]. Biomimetic self-healing mechanisms are still in their infancy, with mimicry of tissue bruising, blood clotting and tailoring of healing networks all being studied. [ ].
An example of a bioinspired self-healing concept is the fusion of broken surfaces by reversible cross-linking polymers. Under controlled load fracture propagation and heat application of up to 150 °C, a healing efficiency of 57% of the original fracture toughness has been achieved in a thermally reversible polymeric material [ ]. Other examples include healing in polymeric material by adding a second solid-state polymer phase [ ] and a two-phase solid-state repairable polymer [ ]. These systems, although offering self-healing abilities, may be considered impractical as they require external intervention, such as a heat source, to activate the healing [ ].
Another approach involves self-healing nanoparticles dispersed in polymer films to be released at a crack site (resembling blood clotting). An computer simulation has been used to model the self-healing efficiency, which could potentially reach 75–100% recovery of mechanical properties of the composites [ ]. This system is considered relevant to biomedical engineering, optical communication and display technologies [ ]. A later work, involving multilayer composites for use in microelectronic and bio-engineering applications, employed fluorescent nanoparticles with ligands (bonding molecules) to control the movement of the nanoparticles to the crack site through a microelectronic film layer [ ].
The third area of study involves self-healing hollow fibres and microcapsules. Microcapsules of dicyclopentadiene (DCPD) monomer in poly urea-formaldehyde (PUF) shells are dispersed within a polymer host and rupture when subjected to a load that causes crack propagation. The healing agents (DCPC) will draw along the fissure line where it encounters a chemical catalyst (usually ruthenium based ‘Grubbs’) incorporated in the polymer matrix. This initiates polymerization and healing occurs [ , , , , ] ( Fig. 4 ). However, DCPD is no longer in use in dental materials. This is perhaps due to biocompatibility complications, probable toxicity of DCPD and Grubb’s catalyst, in addition to the high cost of the material [ , ].

Self-healing dental composites (SHDC) with PUF microcapsules of triethylene glycol dimethacrylate (TEGDMA) monomer and N , N -dihydroxyethyl- p -toluidine (DHEPT) amine accelerator (both as a healing agent) with a benzoyl peroxide (BPO) catalyst incorporated into the composite mixture have been developed. Self-healing properties and fracture toughness (K IC ) recovery of approximately 65% after composite fracture have been observed [ ].
NIR spectroscopy has shown that TEGDMA-DHEPT microcapsules crushed with BPO initiator powder by manual spatulation were polymerized, and after 24 h in an FT-IR spectroscopy the degree of conversion was around 67.2% [ ]. This is in agreement with typical dimethacrylate degree of conversion values which typically range from 55 to 75% [ ]. Thermal analysis reveals that PUF shells of TEGDMA-DHEPT microcapsules could be chemically stable at 150 °C, which is considered a good thermal stability for dental applications [ ]. TEGDMA-DHEPT microcapsules within resin composites show no toxicity during human fibroblast cytotoxicity testing in vitro , hence incorporation of microcapsules into resins does not significantly compromise cell viability [ ].
It has also been shown that different resin monomers can be encapsulated in PUF microcapsules such as TMPET, UDMA, and Bis-GMA or a combination of these monomers and amine activator 4,4′-methylene-bis( N , N -dimethylaniline) (MBDMA). The catalyst BPO and phenyl acetate solution have also been encapsulated and dispersed within dental resin composites. This demonstrated a successful self-healing capability for specimens in water at 37 °C, with a healing performance of around 40% recovery of the virgin fracture toughness [ ].
Different types of healing agents within the core materials of microcapsules have been studied, including three different solvents (chlorobenzene, phenylacetate and ethyl phenylacetate), plus two reactive epoxy resins (diglycidyl ether of bisphenol-F (DGEBF Epon 862 resin) and diglycidyl ether of bisphenol-A (DGEBA Epon 828 resin)). The thermal stability of this type of microcapsule ranged from 150 to 180 °C, due to differences the in boiling temperatures of the encapsulated solvents [ ]. Nanocapsules without a catalyst in the resin have been developed, containing polyurethane (PU) microcapsules of TEGDMA which were then added to dental adhesives to improve the bond strength to dentine tissues. However, these particular nanocapsules have not demonstrated a healing potential since they have no catalyst for polymerization [ ].
A promising strategy in SHDC studied by a number of researchers involves the introduction of a healing powder (strontium fluoroaluminosilicate particles) into the composite along with a healing agent (aqueous solution of polyacrylic acids) encapsulated in silanized silica microcapsules. This concept has a unique mechanism of action as it forms reparative glass ionomer cement (GIC) within the crack when microcapsules are ruptured [ , , ] ( Fig. 5 ).

Self-healing microcapsules can be easily dispersed within a resin matrix. However, there are some disadvantages related to the use microcapsules, such as the necessity of incorporating a catalyst within the resin matrix to initiate polymerization, and the need for microcapsules to rupture when a load is applied. This rupturing is highly dependent on shell thickness and the surface morphology (roughness) of microcapsules that facilitates retention to the resin matrix [ ]. Good catalyst distribution can offer uniform healing, however limited amount of healing agents within microcapsules, and formation of voids when microcapsules empty are downsides of microcapsule self-healing systems [ ]. Other problems arise in fibre-reinforced resin composites; the size of microcapsules (typically 10−100 μm) can disrupt fibre architecture [ ].
Self-healing hollow fibres that act similarly to blood vessels in a natural system have been explored in various engineering materials. A recent work studied the ability to form a ‘bruise’ within a self-healing hollow fibre resin composite, described as ‘bleeding composite’. The author of this project designed a visual damage enhancement method which illustrates the ‘bleeding action’ of an UV fluorescent dye (resembling healing liquid) flow to a crack site and restore mechanical properties of the material [ , ] ( Fig. 6 ). The downsides are the fact that self-healing hollow fibres are of large diameter compared to reinforcement fibres, and the necessity for the fibres to contain low-viscosity healing agents to promote recovery and damage infusion [ ].

4.2
Methods of encapsulation
The procedure of encapsulation has been outlined by many scholars since 1980s; a basic review of in situ encapsulation technique has been reported [ ]. Microencapsulation can be achieved by in situ polymerization of a urea-formaldehyde (UF) in an oil-in-water (O/W) emulsion. This was documented by Brown et al. [ ] then adopted by other researchers with a few procedural modifications.
Shell-forming materials for microcapsules involve urea, formaldehyde, ammonium chloride and resorcinol. All combine together to form a solid capsular shell of poly urea-formaldehyde (PUF). A copolymer such as ethylene-maleic anhydride (EMA) is required (typically 2.5% EMA in aqueous solution) to act as a surfactant which forms an O/W emulsion, wherein oil is the healing liquid [ , , , , , ]. Ammonium chloride is used to catalyse the reaction of urea with formaldehyde to form PUF shells at the oil–water interface, and the use of resorcinol in the reaction is to enhance shell rigidity [ ]. Another way to improve the shell strength is to replace part of the urea with melamine, resulting in a melamine-modified urea-formaldehyde polymer shell, which is known to have a higher bond strength and enhanced microcapsules properties, particularly adhesion to dental resin composite matrix upon microcapsule dispersion [ ]. Different shell materials have also been used: silica microcapsules created by a silica condensation method [ , , ] and polyurethane (PU) nanocapsules by in situ polymerization [ ].
During synthesis of PUF microcapsules, measured amounts of distilled water and surfactant plus shell-forming materials are all mixed in a flask with continuous stirring. The mixture pH is usually adjusted to reach 3–3.5 by drop-wise addition using pH regulators such as sodium hydroxide (NaOH) and/or hydrochloric acid (HCL) [ , , ].
Different healing agents (core material) encapsulated within PUF shells have been reported, with varying materials (monomers) and/or solvents used according to current literature. Noticeably, most of the studies of neat epoxy resin involved DCPD monomer in PUF microcapsules [ , , , , ]. Other studies involving dental resin-based composites described the use of TEGDMA monomer with 1 wt% DHEPT amine as a healing agent in PUF microcapsules [ , ]. One study involves encapsulating Bis-GMA, UDMA, and TMPET or a mixture of these monomers with 0.5 wt% MBDMA amine as a healing agent in PUF microcapsules [ ]. In addition, catalyst PUF microcapsules were also synthesized which contained 90.1 wt% BPO catalyst and 9.9 wt% phenyl acetate mixture solution [ ]. An alternative approach has been described that involves incorporating an aqueous solution of polyacrylic acid in silica microcapsules [ , , ].
Typically, the encapsulation procedure is performed with an external heat source to optimize in situ polymerization reactions. The mixed solution should be agitated throughout the entire encapsulation procedure by magnetic stirring [ , ], mechanical stirring [ , , ] and/or sonication [ , , ]. The agitation speed plays an important role in capsular diameter: the higher the speed of agitation the smaller the diameter of the microcapsules [ , , ]. Nanocapsules usually need a higher speed of agitation using mechanical stirring with a sonication horn used for a short duration (usually less than 5 min) [ , , ]. It has been found that microcapsule diameter, along with shell surface roughness, has a significant effect on capsular rupture and healing performance within self-healing composites [ , ].
The encapsulation procedure, at a target temperature of 55 °C, continues with agitation for 4 h. A suspension of microcapsules will be formed. Once cooled to ambient temperature, filtration and air-drying should follow [ , , , , , ]. The formed suspension of microcapsules can be separated under vacuum filtration with continuous rinsing with deionized water [ , , , ]. Some studies have used solvents in filtration such as acetone [ ], ethanol [ ] or methanol [ ] for rinsing, and others have included a drying agent such as anhydrous magnesium sulphate [ ]. The purpose of rinsing with these solvents is to eliminate excess EMA surfactant from the microcapsule suspension [ ]. However, the use of a solvent is controversial as it may cause capsular surface changes and damage the PUF shells. The harvested microcapsules require air-drying for 24−48 h [ , , ]. This will result in a free-flowing white powder of microcapsules. Other methods have been used to aid separation of microcapsules in order to obtain a free-flowing powder. Methods include centrifugation and sedimentation with continuous rinsing with deionized water, removing the water after each cycle then spray-drying [ ].
Encapsulation procedures vary from one study to another; differences can be found in shell-forming materials, core-forming materials, pH of the mixture, speed of stirring, time and temperature of the process, and filtration. However, the final product of microcapsules should have similar properties. Unfortunately, the filtration procedure is rarely elaborated upon in the literature; and should receive more attention in order to standardise the quality of production. Further attention is also needed regarding storage condition recommendations to limit healing agent leakage from the microcapsules.
4.2.1
Microcapsules silanization
Self-healing microcapsules can be treated with silane coupling agent, which facilitates a strong surface binding within the composite methacrylate resinous matrix, aids microcapsule rupture when composites are subjected to damage stimuli (fracture) [ ], and improves the mechanical properties of the self-healing composite [ ].
Based on SEM analysis, silica microcapsules (polyacrylic acid healing liquid) treated with a methacrylate silane (MA-silane) 3-methacryloxypropyltrimethoxysilane [ , ] demonstrate a rupture rate of approximately 75% of microcapsules at the fracture surface of the composite, compared to only 15% of unsilanized microcapsules [ ]. Another study compared two saline coupling agents: MA-silane linked by covalent bonds, and bishydroxybutyl tetramethylsilane (OH-silane) connected by a H-bond forming hydroxyl silane to the resin matrix. The study found MA-silane self-healing dental composites had nearly five times more microcapsules rupturing compared to OH-silane [ ].
The effect on interfacial interactions of the addition of 3-aminopropyltriethoxysilane coupling agent (KH550) to PUF microcapsules has been explored [ ]. The X-ray photoelectron spectra (XPS) analysis of the interfacial action, chemical bond and hydrogen bond revealed three types of chemical bonding that strongly binds to PUF microcapsules surface. The SEM revealed a thin layer formed on the microcapsule surface. The interfacial adhesion performance of the silanized microcapsules to the epoxy composite improved drastically in comparison to the unsilanized microcapsules [ ] ( Fig. 7 ).
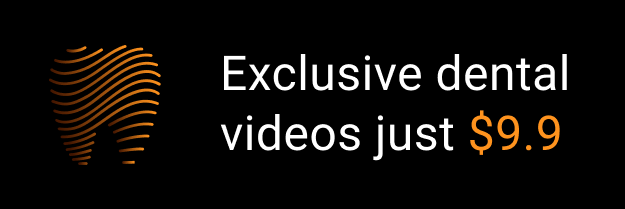