Biological Mechanisms in Orthodontic Tooth Movement
Sunil Kapila, Gregory J. King
The goal of this chapter is to examine the relationships between orthodontic biomechanics and the underlying biological processes. The topics discussed include the factors affecting the rate of tooth movement, anchorage considerations, causes of relapse, and root resorption. All relevant biological principles underlying orthodontic tooth movement can be characterized as tissue remodeling. The process of orthodontic tooth movement is a resultant dynamic change in the shapes and composition of the investing bone and soft tissues. The dental and peridental tissues (dentin, cementum, periodontal ligament [PDL], and alveolar bone) all have active reparative mechanisms and will adapt under the normal forces of orthodontic appliances. At the most basic level, extrinsic forces set up localized areas of “pressure” and “tension” in the tissues adjacent to teeth and the subsequent responses satisfy the principles of Wolff’s law of bone remodeling.1
When orthodontists use fixed appliances to apply force on teeth, predictable tooth movement is anticipated. This is accompanied by transiently increased tooth mobility and, occasionally, radiographic evidence of mild root resorption. Experienced clinicians also expect a certain amount of relapse to occur following orthodontic treatment. Other types of natural tooth migration commonly encountered are eruption of primary and succedaneous teeth as well as mesial or distal drifting of teeth. These physiological processes are not necessarily stimulated by biomechanical signals.
In rare instances teeth fail to erupt or move in response to forces (i.e., ankylosis). Each of these common clinical findings can be explained with a better understanding of the underlying biological principles that determine tooth movement.
Tooth Movement
Clinical Responses
Kinetics of Orthodontic Tooth Movement
From a clinical perspective orthodontic tooth movement has three distinct phases: (1) displacement phase, (2) delay phase, and (3) acceleration and linear phase (Fig. 5-1).
Figure 5-1 Phases of orthodontic tooth movement. The classic curve has three phases: an initial displacement that reflects the viscoelastic properties of the tooth supporting structures; a delay or lag period characterized by no movement; and a postlag phase with linear tooth movement. Minimal tooth movement occurs during the first two phases and most of the tooth movement occurs during the acceleration and linear phases, when alveolar bone remodeling occurs. The timeline is approximate, with considerable individual variation due to mechanical and biological differences.
Displacement Phase.
The initial reaction of a tooth following force application is almost instantaneous (within a fraction of a second) and reflects the immediate movement of the tooth within the viscoelastic PDL cradle. These movements are generally predictable by biophysical principles and typically do not involve extensive amounts of tissue remodeling or deformation of the investing alveolar bone.2 The fluid compartments within the PDL play an important role in the transmission and damping of forces acting on teeth.3 The magnitude of the displacement response is also dependent on root length and alveolar bone height, which are factors that determine the location of a tooth’s center of resistance and center of rotation (see Chapter 4).4,5 For example, loss of alveolar bone results in a more apically positioned center of resistance, which affects the nature of both the initial displacement and net tooth movement (Fig. 5-2). Age is another factor affecting displacement. Young’s modulus of the PDL has been shown to be greater in adults than in adolescents and this difference in biomechanical properties correlates to an equivalent or somewhat increased stress level in the PDL in adults. It is suggested that this might reduce the biological response of the PDL and thus delay tooth movement in adults.6 The displacement capacity of a tooth can change even within the same individual; the elasticity of the PDL and alveolar bone has the potential to be substantially reduced at the end of tooth movement.7
Figure 5-2 Degree of freedom within the viscoelastic periodontal ligament (PDL) apparatus (displacement) is affected by root lengths and alveolar bone heights. A, Varying root length (L) will cause shifts in the positions of the distance of the center of rotation (CROT) to the cervix (a) and the distance of the center of resistance (CRES) to the cervix (x). B, Alveolar bone height changes can affect CROT and CRES. (a, distance of the CROT to the cervix; a′, distance of the CROT to the alveolar crest; L, average root length; L′, varying alveolar bone heights; x, distance of the CRES to the cervix; x′, distance of the CRES to the alveolar crest.) Ultimately the patterns of tooth displacement will be determined by the change in the position of CRES produced by changes in alveolar bone height or root length. (Modified with permission from Tanne K, Nagataki T, Inoue Y, Sakuda M, Burstone CJ. Patterns of initial tooth displacements associated with various root lengths and alveolar bone heights. Am J Orthod Dentofacial Orthop. 1991;100:66–71.)
Delay Phase.
The second phase of the orthodontic tooth movement cycle is characterized by the absence of clinical movement and is generally referred to as the delay or latency phase. During this period there is no tooth movement but extensive remodeling occurs in all tooth-investing tissues. The absolute amount of force applied is not as relevant as the relative force applied per unit area. Depending on the localized compression of the PDL, there can be either (1) a partial occlusion of the blood vessels in the area or (2) an absolute occlusion of blood vessels when high excessive forces have been applied. In cases of partial blockage, the blood vessels delivering nutrients to the area have the capacity to adapt to the new environment and can undergo angiogenesis to bypass occluded areas. However, complete occlusion of vascular flow leads to temporary necrosis of the immediate area and follows a completely different pathway of tooth movement, which is slower to be initiated, starting after approximately 1 to 2 weeks. In either situation, structural and biochemical changes initiate a cascade of cellular mechanisms required for bone remodeling.
Aging has been shown to substantially affect the proliferative activity of the PDL cells and subsequent tooth movement, particularly during the delay phase.8 However, some studies on molar movement in animal models have shown faster initial tooth movement in young subjects than in adults. Yet once tooth movement had reached the linear phase, the rate of tooth movement became equal in both groups. This indicates that the clinically observed increase in orthodontic treatment time for adults can be attributed primarily to the delay phase prior to the onset of tooth movement but the rate of migration is equally efficient once tooth movement has started.9
Acceleration and Linear Phase.
The third phase of the orthodontic tooth movement cycle is characterized by rapid tooth displacement. Tooth movement is initiated in deference to the adaptation of the supporting PDL and alveolar bone changes. Studies on the bone-resorptive osteoclast response following orthodontic appliance activation indicate that when appliance reactivation occurs during the appearance of reactivation osteoclasts, a second cohort of osteoclasts can be recruited immediately. This causes immediate, significant tooth movement with no greater risk of root resorption.10 The force magnitude directly affects the rate of tooth movement. High forces in excess of 100 g used in conventional orthodontic therapy to retract canine teeth have been shown to produce a lag phase of up to 21 days before tooth movement. Lower forces can induce tooth translation without a lag phase at rates that are still clinically significant.11 The difference in rates of tooth movements can be explained by the different biological responses (frontal resorption versus undermining resorption) discussed later in the chapter. Equally as important as magnitude, however, is the timing of the force application. The force regimen has more influence on the rate of orthodontic tooth movement than the force magnitude.12 Light continuous forces are much more conducive to orthodontic tooth movement because the cell biology system remains in a constantly responsive state. Conversely the application of intermittent forces creates a fluctuating environment of cellular activity and quiescence (Fig. 5-3, A). Additionally, it is recognized that very low forces produce lower rates of tooth movement then higher forces (Fig. 5-3, B) up to a specific optimal threshold. Exceeding this optimal force does not result in substantially greater rates of tooth movement. This threshold may differ between individuals as demonstrated in experiments on beagle dogs where it was noted that 25 cN of force caused greater tooth movement than 10 cN of force in one animal but not in another (Fig. 5-3, B and C).
Figure 5-3 Time displacement curves for premolar tooth movement in a beagle dog experimental model demonstrating that (A) light continuous forces of 25 cN are more effective in tooth movement than light discontinuous forces and that (B) continuous forces of 25 cN produce greater movement than 10 cN in one animal, while (C) in another animal the two forces produce equal amounts of tooth movement, demonstrating individual variation and a plateau effect in the latter animal. (Reproduced with permission from van Leeuwen EJ, Maltha JC, Kuijpers-Jagtman AM. Tooth movement with light continuous and discontinuous forces in beagle dogs. Eur J Oral Sci. 1999;197:468–474.)
Ankylosis
In rare cases a tooth may not move at all, regardless of the amount of external force applied on it. A likely cause of this is a phenomenon known as “ankylosis” where the PDL fibers are conspicuously absent and therefore cannot serve as an intermediary between the root structure and the alveolar bone. The contact point is a direct fusion of the cementum layer to the cortical bone of the tooth socket. Apart from idiopathic ankylosis, the primary cause of ankylosis is extrinsic localized trauma.13 In cases of severe dental trauma, such as avulsion or intrusion, there is injury to the periodontal membrane resulting in a direct fusion of the alveolar bone with the tooth. Consequences of this condition include progressive resorption of the root with replacement by bone (replacement resorption) and arrested growth of the alveolar process in growing patients. Individuals with congenitally missing succedaneous permanent teeth characteristically exhibit infraocclusion and overretention of ankylosed deciduous teeth.14 Partial ankylosis can occur when only limited areas of the teeth are fused to the bone. If these localized regions of bone–tooth attachment can be overcome with sufficient force application, the remainder of the tooth that does have PDL support can proceed toward a normal pattern of tissue remodeling and tooth movement.
Principles of Anchorage in Orthodontics
The biomechanics of orthodontics are not always designed for the purpose of moving teeth. In certain cases the intent of the clinician may be to hold the position of certain teeth in the arch or to use groups of teeth comprising an “anchor unit” to serve as a foundation for pushing or pulling other teeth. Several types of anchorage are used in orthodontics: (1) extraoral anchorage appliances (e.g., headgear) where external skeletal structures are used, (2) intraoral osseointegrated implants and temporary anchorage devices (TADs) that are physically interlocked within the bone and therefore are very stable, and (3) dental anchorage, which is essentially the preparation and consolidation of teeth into units for use in pushing or pulling the rest of the dentition.
Dental anchorage is a term applied to the intentional minimization of migration of specific teeth through the supporting alveolar bone structure. The following section elaborates on dental anchorage since it is based on the premise of biological adaptation to orthodontic forces. Dental anchorage can be increased either by increasing the number of teeth consolidated into the anchor unit or by intentionally angulating specific teeth to better resist movement, or both. In general, teeth with greater root surface area tend to move less when used to move teeth with less root surface area. This occurs because the ability to resist movement is directly related to the periodontal fibers and bone surface area engaged in withstanding tooth movement. When forces are light and distributed over large surface areas, the compression on underlying periodontal structures leads to a partial vascular occlusion of the system and a transient ischemia. Although limited, there is still oxygenation to the area, enabling the microsystem to adapt and recruit new blood vessels for initiation of frontal resorption to occur. Movement of teeth with frontal resorption occurs within 3 to 4 days. However, when hyalinization of bone occurs in areas of periodontal compression during force application, there is a significant retardation of tooth movement while undermining resorption occurs. In this case the resistance to tooth movement is due to complete vascular occlusion in the compression area causing localized necrosis of bone and undermining resorption. When this happens the teeth start moving only after 12 to 15 days of bone remodeling. Therefore anchorage preparation is affected by the magnitude of forces applied, the total root surface area of the teeth upon which the forces are applied, and the angulation of the teeth.
Increasing numbers of adults are seeking orthodontic treatment today and in these cases anchorage becomes a critical concern. Extraoral anchorage appliances are not usually a feasible alternative for these individuals. Therefore the clinician must maximize all available resources, such as the engagement and colligation of second molars (and third molars, if present) into the dental anchorage unit as well as the use of palatal anchorage devices such as the Nance acrylic button appliance. Also in multidisciplinary cases, implants and other fixed restorative devices can and should be incorporated into the treatment plan for use as anchorage units during orthodontics. Finally, the introduction of TADs has provided substantial advantages for anchorage preservation in adults and adolescents alike and has opened up novel approaches to orthodontic biomechanics in complex cases.15
Histological Responses
In general, teeth can move through their investing tissues with or without histological evidence of tissue injury. There is no evidence that the intra-alveolar phase of tooth movement during physiological eruption, drift, or relapse is mediated by pathological processes.16–18 However, most studies of orthodontic tooth movement have described pathological processes at sites of compression, including vascular collapse, compensatory hyperemia, and tissue necrosis. Hyperemic changes are not restricted to the periodontal tissues adjacent to compression but have also been variously described in the adjacent marrow spaces and the dental pulp.
Tooth Movement Without Injury
The most obvious course of physiological tooth movement is the intra-alveolar eruption of teeth. As the crown of a tooth completes mineralization and begins its process of migration through the alveolar bone, it becomes enclosed in a crypt. This crypt is translated bodily by a combined effort of osteoclastic bone resorption along the path of eruption and osteoblastic bone formation on the path that the crown has already taken. The rate-limiting factor of the earliest (intraosseous) stage of tooth eruption is bone resorption and eruption can be accelerated or retarded by the local delivery of factors that alter the rate of osteoclastic activity.19 Certain hormones, such as parathyroid hormone–related protein (PTHrP), have been shown to be crucial in the normal tooth eruption processes and cementogenesis.20,21 Pathological systemic conditions with dysfunctional PTH/PTHrP or their cognate receptors can lead to the prevention of normal tooth eruption and inhibition of normal cementogenesis.
As teeth erupt into the oral cavity, and even throughout life, there is a natural tendency for them to continue moving along the path of least resistance until they encounter an obstacle of resistance. Usually this barrier comes in the form of interproximal contact from an adjacent tooth or occlusal contact from a tooth in the opposing arch. In the absence of this resistance there will be continued mesiodistal tipping or supraeruption, depending on the location of deficient contact. Studies have shown that mesial drifting of a tooth can have clinical significance on its morphological composition. In the process of mesial migration, tensional forces on the distal root surfaces may account for the increased cementum thickness on the distal surfaces of mesially drifted teeth.22 As for supraeruption, in a mouse model where the opposing molar was extracted to induce occlusal hypofunction, histological staining showed that after 15 days of hypofunction the PDL was significantly narrowed and the fibrous nature was very disorganized for a minimum of 30 days and up to 3 months. There was concurrent deposition of disorganized woven bone at the top of the interradicular septa, at the bottom of the sockets, and along the modeling sides.23 Thus it is evident that loading is an integral part of the maintenance of the supporting structures surrounding the tooth.
Tooth Movement with Injury
Necrotic lesions at compression sites in the PDL space have been described in the early literature documenting the histological changes accompanying orthodontic tooth movement.24 These areas are referred to as “hyalinization zones” because of their similarity in appearance to hyaline cartilage. Modern morphological techniques have elucidated that these so-called “hyalinizations” are in fact areas of focal tissue necrosis.25 As long as these lesions persist, orthodontic tooth movement does not occur. This period is coincident with the delay phase of the tooth movement cycle. Specialized phagocytic cells are recruited and migrate to the site to remove these necrotic lesions. These cells remove the injured tissue from the periphery, resulting in the resorption of not only the necrotic soft tissue lesion but also the adjacent alveolar bone and cementum.26
The tissue responses at sites of tension are consistent with those that have been described for other sites where soft tissue separates bone. In addition to the PDL, such sites can be found naturally in the craniofacial complex at sutures and artificially at sites of osteodistraction. Tensile forces are known to initiate an exuberant osteogenic response at these locations, with the first bone being deposited on the stretched soft tissue scaffold (Fig. 5-4). Through remodeling processes new compact bone is eventually deposited at these sites. This so-called consolidation process is slow to occur and therefore tends to lag behind the tissue removal activity that is simultaneously occurring at compression sites. The clinical result is the prevalence of increased mobility in teeth that are actively being treated orthodontically. This difference in timing between tissue removal and osteogenesis also accounts for the need to retain teeth that have recently been moved.
Figure 5-4 Morphological changes on the tension side during orthodontic tooth movement. A, Initial changes are characterized by stretching of the principal periodontal ligament (PDL) fibers, seen here as linear orientation of cell nuclei adjacent to the tooth. B, Later changes show deposition of bone on the stretched PDL fibers, oriented perpendicular to the tooth and socket wall (arrows). Bn, Alveolar bone; T, tooth root. C, The three-dimensional organization of these initial bony spicules can be appreciated with a scanning electron micrograph of the alveolar bone on the socket wall after removal of the tooth and PDL. The micrograph is looking into the socket with the tension socket wall on the right.
In addition to bone remodeling, histological evidence has shown that initial root resorption occurs in the peripheries of the necrotic PDL following orthodontic treatment (Fig. 5-5). This is a result of mononucleated nonclast macrophage-like and fibroblast-like cellular activity.27,28 Minor resorptive lacunae created on the root surface by cementoclasts can be repaired gradually over time. However, heavy forces create extensive defects on the root surface, which exceed reparative capacity and lead to craterlike topography on the root surface and at the apex (Fig. 5-6).
Figure 5-5 Morphological changes on the compression side showing tissue and cellular responses leading to root resorption during orthodontic tooth movement. A, Initial changes are characterized by focal areas of periodontal ligament (PDL) necrosis, so-called hyalinization, seen as the clear area in the PDL running vertically down the center of this micrograph. Areas of vascular congestion can be seen adjacent (pulp, PDL, and marrow spaces) to the necrotic PDL (arrows). B, Later changes show removal of the necrotic PDL and adjacent tissues, including the root cementum and dentin from the periphery by osteoclasts, cementoclasts, and macrophages (arrows). The remaining necrotic PDL is seen as the clear pink area in the lower middle of the micrograph. Vital adjacent PDL can be seen as the highly cellular areas above and below the necrosis. Bn, Alveolar bone; T, tooth root.
Figure 5-6 Tooth injury resulting in root resorption following bone remodeling. A, Apical third of the lingual root surface of the mandibular left bicuspid control tooth demonstrating the absence of resorption. B, Apical resorption and loss of root length association with a multitude of resorption pits over the lingual root surface as a result of 2 weeks’ application of a continuous 10-g intrusive force. Many of the resorption loci have coalesced to form extensive invasive lesions. C, Lingual aspect of a maxillary right bicuspid showing early apical resorption caused by 14 days’ intrusion with 50 g. Original magnifications for A and B are ×20 (bar = 300 mm) and for C is ×40 (bar = 200 mm). (Reproduced with permission from Harry MR, Sims MR. Root resorption in bicuspid intrusion: a scanning electron microscope study. Angle Orthod. 1982;52:235–258.)
Bone Turnover in Orthodontic Tooth Movement
Osteogenesis and Bone Modeling and Remodeling
Bone structure can be changed in three principle ways: (1) osteogenesis, (2) bone modeling, and (3) bone remodeling. Osteogenesis is when bone is formed on soft tissue and generally occurs during embryonic development, the early stages of growth, and healing. There are two major subclassifications: intramembranous ossification and endochondral ossification, in which bone is formed on soft fibrous tissue and, usually, cartilage, respectively. Osteoblasts are a differentiation product from mesenchymal cells and act independently of osteoclasts, resulting in a large potential to create significant amounts of bone.
Modeling is characterized by bone formation on existing bone tissue over extended surface areas for significant periods of time. This type of bone turnover is prevalent during craniofacial growth and development and leads to change in shape of the structure or translation of the surface. For example, the mandibular alveolar process increases in length by resorptive modeling on the anterior surface of the ramus and by formative modeling on its posterior surface. From an orthodontics standpoint, modeling is important in normal growth of the craniofacial structure as well as changes in alveolar size and shape during tooth movement.
Remodeling is a reparative mechanism and involves a series of cellular events that occur cyclically throughout life (Fig. 5-7). It is the only physiological mechanism for maintaining and repairing the structural integrity of bone. The bone remodeling cycle begins with a period referred to as activation that is characterized by the recruitment and activation of osteoclasts at the site to be remodeled. This is followed by a resorptive phase when a “packet” of bone is removed. After a finite amount of time the resorptive process ceases. This phase is termed reversal. Reversal is followed by a formative phase characterized by the recruitment of bone formative cells to the site and the active repair of the defect created during the resorptive phase. Once the cycle is complete, the bone surface returns to a resting state. In healthy adults bone surfaces are primarily in a resting state, although a small fraction of the cell population can be seen to progress through the other phases. Remodeling is important in calcium homeostasis as well as in producing changes in bone matrices that modify the mechanical properties of the bone in response to altered loading. Remodeling and modeling of bone are differentiated by the fact that while osteoblast and osteoclast activities are found on the same site in remodeling, they occur on different sites in modeling, which enables morphological changes in the bone.
Figure 5-7 Cellular quiescence phase and five phases of cellular activity in remodeling of trabecular bone. BSU, Bone structural unit or newly formed bone structure; CL, closed lacunae; HL, Howship lacunae/resorption pit; LC, lining cells; OB, osteoblast; OC, osteoclasts; OS, osteoid; POC, osteoclast precursors.
Cellular and Molecular Mechanisms of Bone Modeling and Remodeling
Skeletal integrity is the result of a dynamic interaction between bone-forming osteoblasts and bone-resorbing osteoclasts. The rate of remodeling is defined primarily by cells of the osteoblast lineage, which in addition to bone formation are also responsible for the activation and recruitment of osteoclast precursors.29–31 The basis of communication between osteoblasts and osteoclasts was unclear until several groups independently identified the presence of an intermediary factor on the surface of osteoblasts that was responsible for the induction of osteoclastogenesis. This factor is a member of the tumor necrosis factor (TNF) superfamily and was termed receptor activator of nuclear factor κB ligand (RANKL).32,33 Binding of RANKL to its cognate receptor, receptor activator of nuclear factor κB (RANK), expressed on the surface of osteoclast progenitor cells, induces osteoclastogenesis and activates osteoclasts (in the presence of macrophage colony-stimulating factor), resulting in increased bone resorption.34,35 However, RANKL also has the potential to bind to osteoprotegerin (OPG), a soluble decoy receptor protein that competitively binds to cell surface membrane–bound RANKL proteins and inhibits RANKL activation of osteoclastogenesis. The interaction of RANKL with OPG therefore decreases bone resorption (Fig. 5-8).36 The ratio of RANKL/OPG expression by osteoblasts is believed to be a key determinant of the rate of recruitment and activation of immature osteoclasts. In dentistry these genes have already been strongly implicated as the causative factors of alveolar bone changes. RANKL and OPG protein production has been detected in human periodontal cells.37 Pathologically, lymphocytes and macrophages in periodontitis tissues show correlations with RANKL protein production and endothelial cells have associations with OPG production.38 From an orthodontic perspective, it is very likely that pressure changes in the microenvironment of the tooth socket may cause up-regulation and down-regulation of the RANKL and OPG genes as a means of modulating protein production and ultimately bone remodeling.
Figure 5-8 Regulation of osteoclastogenesis by osteoblasts. Receptor activator of nuclear factor κB ligand (RANKL) induces immature osteoclast (OC) precursors to differentiate into mature and functional osteoclasts, while osteoprotegerin (OPG) is a decoy receptor that acts as a competitive binding inhibitor of RANKL. Since RANKL is a cell surface–bound receptor protein, cell–cell interaction is required. Macrophage colony-stimulating factor (M-CSF) is also an essential co-factor. Vitamin D3 and various cytokines have been demonstrated to have down-regulatory effects on OPG gene expression and up-regulatory effects on RANKL gene expression. PTH, Parathyroid hormone; PTHRec, PTH receptor; PTHrP, PTH-related protein.
Besides the prominent role of the RANKL/OPG ratio in the regulation of osteoclasts by osteoblasts, the rate of bone remodeling is controlled by other local and systemic mechanisms. Local, or paracrine, mechanisms involve numerous inflammatory cytokines (e.g., interleukins, TNFs, and growth factors) that have biological activities influencing individual phases of the cycle (Fig. 5-9).39 In addition, there is evidence that alterations in the genetic expression of bioactive agents can occur directly on bone cells. Systemic control of bone remodeling occurs through several endocrine mechanisms, including the calciotropic hormones (e.g., parathyroid hormone [PTH] and 1α,25-(OH)2 vitamin D3) and the sex steroids (e.g., estrogen).40–42 These factors act on osteoblasts as an intermediary to regulate osteoblast-osteoclast equilibrium and can either up-regulate or down-regulate a cascade of downstream signaling pa/>
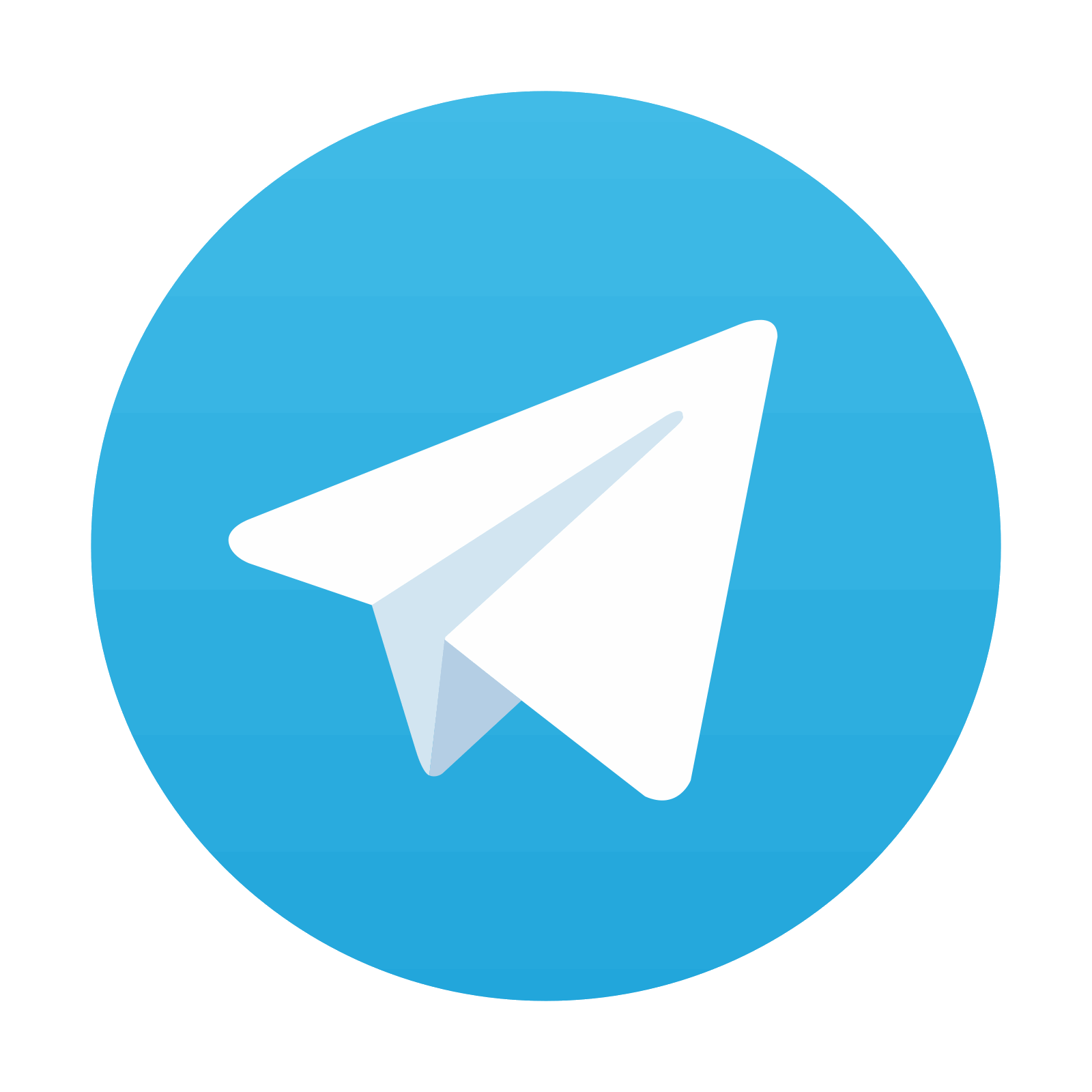
Stay updated, free dental videos. Join our Telegram channel

VIDEdental - Online dental courses
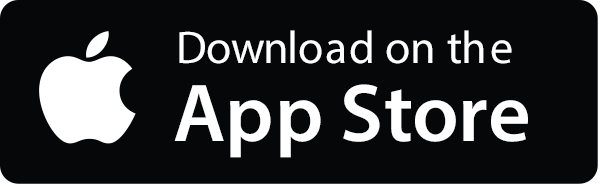
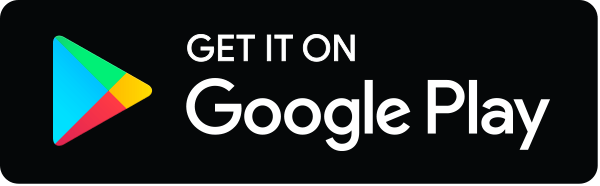