Biomaterials
The science of biomaterials for restorative dentistry is derived from the science of materials. Biomaterials include synthetic and tissue-engineered biomaterials. Synthetic biomaterials can be organized in terms of four categories of materials, with four categories of structural considerations that govern their properties and four categories of general properties. For each of these categories, a rich basis of materials science definitions exists. This information is presented in greater depth in biomaterials textbooks, but it is reviewed here for reference during discussions in other parts of this book.1–24
Tissue-engineered biomaterials have existed approximately 40 years as simple biomimetic structures. Since the publication of the human genome and the explosion of post-genomic efforts, however, tissue engineering has gained substantial momentum. Replacement of human tissue with new tissue can be accomplished by generating replacements outside of the body or in situ in the body. In each case, the key elements are described as the tissue engineering triad of scaffolds, cells, and signals (Online Fig. 18-1).25,26 Scaffolds can be produced synthetically or derived naturally. Typical synthetic scaffolds include polylactic acid–polyglycolic acid (PLA-PGA) co-polymers, which have the advantage of being biodegradable and biologically resorbable, and naturally derived scaffolds include reconstituted collagen from a variety of nonhuman sources. At present, mature differentiated mammalian cells (e.g., osteoblasts) are placed, or seeded, on scaffolds.27–29 The use of undifferentiated stem cells in tissue-engineered constructs also offers great promise.30 Signals such as bone morphogenetic proteins are crucial in orchestrating the development of the normal biologic architecture of a tissue. These signals can be collected from other environments and added or generated by growing and developing cells. The entire process can be staged outside of the body and implanted, conducted in a biologic setting and replanted to the final location, or managed in situ. Ex vivo work has been facilitated by using inkjet deposition techniques to build three-dimensional structures.31–33
Despite optimism about this process and its ultimate potential, myriad problems need to be managed and barriers are yet to be overcome. Although the process may sound simple, reliable control of these systems is daunting. Many other new technologies may become part of this science. Nano-engineering, in combination with evolving knowledge about self-assembling systems, offers the possibility of using molecular scale processes to create building blocks for in situ engineering of scaffolds and chemical triggers for controlling the signaling of cells. Normal biologic processes involve self-assembly of tissues but are high-energy events. Low-energy self-assembly is a new science and offers help for many of the tissue engineering steps.29,30
Review of Materials Science Definitions
Material Categories
Metals
A metal is an element that diffusely shares valence electrons among all of the atoms in the solid, instead of forming local ionic or covalent bonds. A metal alloy is an intentional mixture of metallic elements that occurs in a chemically intimate manner. As a result of mixing, the elements may be completely soluble (e.g., gold–copper [Au-Cu]) or may be only partially soluble (e.g., silver–tin [Ag-Sn]), producing more than one phase. Metallic systems are almost exclusively crystalline, and most exist as polycrystalline solids. The individual crystals, or grains, are generally microscopic. Grains may be all the same composition (single-phase) or several different phases (multiple-phase). Different phases represent locally different chemical compositions. In metal alloys, no phase (or crystal or grain) ever represents a pure metallic element (Online Fig. 18-2). The distribution of phases is influenced by the thermal and mechanical histories of the solid, allowing a wide range of properties to be developed from a single overall composition. The periodic table consists mostly of metallic elements. A wide range of potential metallurgic systems exists.
Ceramics
Ceramics are chemically intimate mixtures of metallic and non-metallic elements, which allow ionic (potassium oxide [K2O]) bonding, covalent (silicon dioxide [SiO2]) bonding, or both to occur. In the periodic table, only a few elements such as carbon, oxygen, nitrogen, hydrogen, and chlorine, are nonmetallic. The most common ceramics in dentistry are semi-crystalline (Online Fig. 18-3, A) and are chemical mixtures of three main metallic oxides (SiO2, aluminum oxide [Al2O3], K2O) (see Online Fig. 18-3, B). Ceramics also may result from corrosion of metals (iron oxide [Fe2O3], tin oxide [SnO], silver sulfide [Ag2S]).
Ceramics may be classified on the basis of (1) being crystalline, non-crystalline, or both; (2) being predominantly based on silica (SiO2) and called silicates; (3) being predominantly formed by metal reactions with oxygen and called oxides; or (4) involving relatively simple parent structures (main structures) or highly substituted ones (derivative structures). Most ceramics are semi-crystalline, silicates, oxides, and derivative structures (see Online Fig. 18-3, B). Simple ceramic structures are more often ionically bonded. More complicated structures generally involve combinations of ionic and covalent bonding.
Polymers
Polymers are long molecules composed principally of non-metallic elements (e.g., carbon [C], oxygen [O], nitrogen [N], hydrogen [H]) that are chemically bonded by covalent bonds. Their principal distinction from other common organic materials is their large size and molecular weight. The process of forming a polymer from identifiable subunits, monomers, is called polymerization (Online Fig. 18-4). Monomer means “one unit”; polymer means “many units.”
Acrylic monomers are used widely in dentistry and undergo chain-reaction polymerization. The stages of chain-reaction polymerization (see Online Fig. 18-4, A) include (1) activation (production of free radicals), (2) initiation (free radical combination with a monomer unit to create the beginning of a growing chain), (3) propagation (continued addition of monomer units), and (4) termination (cancellation of the growing chain end by any one of several possible events). The reaction kinetics of any step may be complex and may be influenced by many variables such as temperature, extent of reaction, or method of initiation. Accelerators (chemical, light, or heat) may be used to increase the rate of activation. Inhibitors or retarders (chemical) may be added to consume newly formed free radicals and prevent or postpone initiation. When chain-reaction polymerization has started, the process may proceed at extremely high speeds, producing extensive release of heat. Methyl methacrylate monomers combine to form polymer at a rate of one million units per second.
Composites
Composites can be described as a dispersed (filler) phase mixed into a continuous (matrix) phase (Online Fig. 18-5). The matrix phase is generally the phase that is transiently fluid during the manipulation or placement of materials. It also is the phase that tends to have the least desirable properties in the mixture. As a general rule, minimizing the matrix of any system produces materials with more desirable clinical properties. For a composite to distribute energy within the system to all of the phases, it is important that the dispersed phase be bonded effectively to the continuous phase.
Material Properties
Physical Properties
Physical properties involve reversible interactions of a material with its environment. A few common physical properties are reviewed here with respect to important dental situations. Metals, ceramics, polymers, and composites have different types and numbers of bonds. During temperature changes, these materials respond differently. During temperature increases, more frequent atomic motions stretch the bonds and produce net expansion. During temperature decreases, solids undergo contraction. The relative rate of change is called the coefficient of thermal expansion (or contraction). If it is referenced to a single dimension, it is called the linear coefficient of thermal expansion (LCTE), symbolized by the Greek letter alpha (α). The LCTE is expressed in units of “inch/inch/°F,” “cm/cm/°C,” or “ppm/°C.” Because the rate of change is small, the actual value is typically a multiple of 10−6 cm/cm/°C and is reduced to ppm/°C. Ceramics typically have an LCTE of 1 to 15 ppm/°C. Metals typically have values of 10 to 30 ppm/°C. Polymers typically have values of 30 to 600 ppm/°C. The LCTE of tooth structure is approximately 9 to 11 ppm/°C. It is important that the LCTE of a restorative material be as near that of tooth structure as possible. Online Table 18-1 presents examples of values for biomaterials.
Online Table 18-1
Linear Coefficients of Thermal Expansion
Biomaterials/Structures | LCTE (ppm/°C) |
Aluminous dental porcelain | 4 |
Alumina | 6.5–8 |
In-Ceram | 8–10 |
CP-titanium | 8–9 |
Traditional dental cements | 8–10 |
Tooth structure | 9–11 |
Stainless steel | 11 |
PFM ceramics | 14 |
PFM alloys | 14 |
Gold foil | 14–15 |
Gold casting alloys | 16–18 |
Co-Cr alloys | 18–20 |
Hybrid glass ionomers | 20–25 |
Dental amalgam | 25 |
Packable composites | 28–35 |
Anterior and flowable composites | 35–50 |
Composite cements | 40 |
PMMA direct-filling resins | 72–83 |
Dental wax | 260–600 |
From Bayne SC, Thompson JY: Biomaterials science, ed 7, Chapel Hill, NC, 2000, Brightstar.
One of the consequences of thermal expansion and contraction differences between a restorative material and adjacent tooth structure is percolation. This process is typified by an intracoronal amalgam restoration. During cooling, amalgam contracts faster than the tooth structure and recedes from the preparation wall, allowing the ingress of oral fluids. During subsequent expansion, the fluid is expressed. Cyclic ingress and egress of fluids at the restoration margin is called percolation (schematically presented in Online Fig. 18-6).
Other important physical properties involve heat flow through materials. Enamel and dentin are composed primarily of finely packed ceramic crystals (i.e., hydroxyapatite, Ca10[PO4]6[OH]2) that make those structures act as thermal insulators. If the tooth structure is replaced by a metallic restoration, which tends to be a thermal conductor, it may be important to provide thermal insulation to protect the dental pulp from rapid increases or decreases in temperature in the mouth. Generally, dental cements that may be used as bases under metallic restorations act as insulators. An advantage of a composite is low thermal conductivity. Composites do not need liners and bases to provide thermal insulation. Heat flow through a material is measured in terms of either the relative rate of heat conduction (thermal conductivity) or the amount of heat conduction per unit time (thermal diffusivity). Thermal diffusivity is the more important property because it determines the amount of heat flow per unit time toward the pulp through a restoration. The dental pulp can withstand small temperature changes (37-42°C) for relatively short periods (30–60 seconds) without any permanent damage.5,34,35 Under most circumstances, the microcirculation of the pulp transports the heat entering the pulp away to other parts of the body, where it is dissipated easily. Extreme temperature changes or extended times of exposure to high temperatures cause pulpal changes, however.
Optical properties of bulk materials include interactions with electromagnetic radiation (e.g., visible light) that involve reflection, refraction, absorption (and fluorescence), or transmission (Online Fig. 18-7). The radiation typically involves different intensities for different wavelengths (or energies) over the range of interest (spectrum). Any of these interactive events can be measured using a relative scale or an absolute scale. When the electromagnetic radiation is visible light, the amount of reflection can be measured in relative terms as gloss or in absolute terms as percent reflection. Visible light absorption can be measured in absolute terms as percent absorption (or transmission) for every wavelength (in the visible spectrum). Color is a perception by an observer of the distribution of wavelengths. The same color sensation may be produced by different absorption spectra (metamerism). An individual’s eye is capable of sensing dominant wavelength, luminous reflectance (intensity), and excitation purity. Variations among individuals’ abilities to sense these characteristics give rise to varying perceptions of color.
Color measurement techniques do not measure these quantities directly. Color traditionally has been measured using the Munsell color system in terms of hue, value, and chroma. These terms correspond approximately to wavelength, intensity, and purity. The relationships of these quantities are represented schematically in Online Figure 18-8. Shade guides for matching restorative biomaterials to the tooth structure are based on this system of describing color. The quality of color also is measured by the Commission Internationale de l’Eclairage system as tristimulus values and reported as color differences (ΔL*, Δa*, and Δb*) compared with standard conditions.
A wet tooth that is isolated from the wetting by saliva soon has a transient whiter appearance. Most of this shade change is the effect of loosely bound water lost from subsurface enamel (by dehydration) between hydroxyapatite crystals. This increases the internal scattering of light, with much of it reflected back to the observer (see internal reflection in Online Fig. 18-7). This probably explains why it takes 15 to 20 minutes for the isolated tooth to develop the whiter appearance and 30 minutes or more for it to regain its original appearance after isolation is terminated. Larmas et al showed that 0.8% to 1% by weight of pulverized moist enamel is exchangeable water and that it can be removed at 4% relative humidity and 20°C.36 Loosely bound water also provides channels for diffusion through enamel of ions and molecules. The direction of radiation may be perturbed as it crosses an interface from a medium of one type of optical character to another. Refractive index is the angle of changed path for a standard wavelength of light energy under standard conditions.
Another group of physical properties comprises surface properties. Surfaces are important because all restorative biomaterials meet and interact with the tooth structure at a surface. Also, all dental surfaces interact with intraoral constituents such as saliva and bacteria. Changing a material’s surface properties can mitigate the extent of that interaction. The type of interaction between two materials at an interface is defined as the energy of interaction, and this is conveniently measured for a liquid interacting with a solid under a standard set of conditions as the contact angle (θ). The contact angle is the angle a drop of liquid makes with the surface on which it rests (Online Fig. 18-9, A). This angle is the result of an equilibrium between the surface tensions of the liquid–gas interface (γLG), solid–gas interface (γSG), and solid–liquid interface (γSL). These relationships can be expressed as an equation (see Online Fig. 18-9, A). If the energy difference of the two materials in contact is large, they have a large contact angle. If the energy difference is very small, the contact angle is low, and the liquid appears to wet the solid by spreading. Wetting is a qualitative description of the contact angle. Good wetting, or spreading, represents a low contact angle. Partial (poor) wetting describes a contact angle approaching 90 degrees. Nonwetting is a contact angle approaching 180 degrees (see Online Fig. 18-4, B).
Mechanical Properties
The mechanical properties of a material describe its response to loading. Although most clinical situations involve complicated three-dimensional loading situations, it is common simply to describe the external load in terms of a single dimension (direction) as compression, tension, or shear. Combinations of these can produce torsion (twisting) or flexion (transverse bending). These modes of loading are represented schematically in Online Figure 18-10, with respect to a simple cylinder and a mesio-occlusal amalgam restoration. For testing purposes, it is often impossible to grip and pull a specimen in tension without introducing other, more complicated stresses at the same time. To circumvent problems for tensile testing of cylinders, it is possible to compress the sides of a cylinder and introduce stresses equivalent to tension. This variation of tension is called diametral tension (or diametral compression).
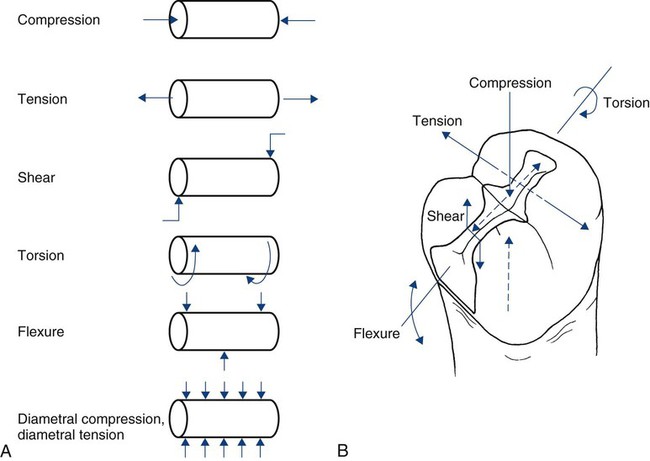
When a load is applied, the structure undergoes deformation as its bonds are compressed, stretched, or sheared. The load-deformation characteristics are useful only if the absolute size and geometry of the structure involved are known. It is typical to normalize load and deformation (in one dimension) as stress and strain. Stress (abbreviated σ) is load per unit of cross-sectional area (within the material). It is expressed in units of load per area (lb/in2 = psi, or N/mm2 = MPa). Strain (abbreviated ε) is deformation (ΔL) per unit of length (L). It is expressed in units of length per length (inch/inch, or cm/cm), which is a dimensionless parameter. A schematic summary is presented in Online Figure 18-11. During loading, bonds generally are not compressed as easily as they are stretched. Materials resist compression more readily and are said to be stronger in compression than in tension. Materials have different properties under different directions of loading. It is important to determine what the clinical direction of loading is before assessing the mechanical property of interest.
As loading continues, the structure becomes deformed. At first, this deformation (or strain) is completely reversible (elastic strain). Increased loading finally produces some irreversible strain as well (plastic strain), however, which causes permanent deformation. The point of onset of plastic strain is called the elastic limit (proportional limit, yield point). This point is indicated on the stress–strain diagram (see Online Fig. 18-11) as the point at which the straight line starts to become curved. Continuing plastic strain ultimately leads to failure by fracture. The highest stress before fracture is the ultimate strength (see Online Fig. 18-11, C). The total plastic tensile strain at fracture is called elongation; this also may be expressed as the percent elongation. Materials that undergo extensive plastic deformation before fracture are called ductile (in tension) or malleable (in compression). Materials that undergo very little plastic deformation are called brittle.
It is often convenient to determine the elastic limit in a relative manner by comparing the onset of plastic deformation of different materials using scratch or indentation tests, called hardness tests. The Mohs hardness scale ranks scratch resistance of a material compared with a range of standard materials (Online Table 18-2). Rockwell, Brinell, and Knoop hardness tests employ indenters instead. The energy that a material can absorb before the onset of any plastic deformation is called its resilience (see Online Fig. 18-11, C) and is described as the area under the stress–strain curve up to the elastic limit. The total energy absorbed to the point of fracture is called toughness and is related to the entire area under the stress–strain curve (see Online Fig. 18-11, C).
Online Table 18-2
Mohs Hardness/Reference Material | Mohs Hardness/Materials Examples |
10/Diamond (C) | 9–10/Silicon carbide (SiC) |
9.5/Tungsten carbide (WC) | |
9/Corundum (Al2O3) | 8–9/Chrysoberyl (FeAl2O4) |
8/Zirconia (ZrO2) | |
8/Topaz (Al2SiO4(FOH)2) | 7–8/Tool steels |
7/Quartz (SiO2) | 6–7/Garnet |
6.5–7.5/Feldspathic | |
6–7/Porcelain | |
6/Pumice | |
6/Orthoclase (KAlSi3O8) | 5–6/Dental enamel [(Ca6(PO4)10(OH)2)] |
5–5.5/Dental composite | |
5/Apatite (Ca5(PO4)3(OH) | 4–5/Low-carbon steels |
4–4.5/Platinum | |
4–5/Amalgam | |
4/Fluorite (CaF2) | 3–4/Dentin |
3.5/Copper penny | |
3–4/Plastic | |
3/Calcite (CaCO3) | 2–3/Copper (Cu), silver (Ag), gold (Au) |
2/Gypsum (CaSO4-2H2O) | 1–2/Ice |
1/Talc (Mg3Si4O10(OH)2) |
During loading, for all practical purposes, the strain below the elastic limit is all elastic strain. The amount of plastic strain is infinitesimal—so small that it is ignored. During multiple cycles, these very small amounts of plastic strain begin to accrue. After millions of cycles, the total plastic strain accumulated at low stress levels may be sufficient to represent the strain required to produce fracture. This process of multiple cycling at low stresses is called fatigue (Online Fig. 18-12, A). A standard engineering design limit for dental restorative materials is approximately 10 million cycles (or approximately 10 years of intraoral service). A rule of thumb is that materials on working surfaces of teeth are mechanically cycled approximately one million times per year on average. The curve correlating cyclic stress levels (S) to the number of cycles to failure (N) is called fatigue curve (S-N curve). These curves have been determined only for a few biomaterials because conducting the tests requires such a long time.37 The compressive fatigue curves for Tytin and Dispersalloy amalgams are shown as part of Online Figure 18-12, B.
Mechanical properties can be used to describe the behavior of liquids and solids. As the temperature of a solid is increased, its stress–strain curve shifts downward and to the right. At the melting point, the stress–strain curve is a horizontal line lying at zero stress along the strain axis. Rather than examining the stress–strain behavior of liquids, it is more meaningful to examine the shear stress (τ) versus shear strain rate (γ). Well-behaved liquids (newtonian behavior) form a straight line (Online Fig. 18-13). Departures may occur that produce lines curving down (pseudoplastic behavior) or curving up (dilatant behavior). In some cases, the starting point of the line is shifted up along the shear stress axis, representing a material that does not start to flow until a critical shear stress has been reached (Bingham body behavior). Pseudoplastic and Bingham body behaviors are typical for biomaterials. The lines on these diagrams are described by a relatively simple equation, ηn = τ/γ, which is similar to the equation for elastic modulus, E = σ/ε. The term η, or viscosity, is the resistance to flow or stiffness of the liquid. As the temperature is increased above the melting point, the viscosity behavior tips down and toward the right. A 37% phosphoric acid solution gel used for etching displays pseudoplastic Bingham body behavior. It does not flow until a critical shear stress is exceeded, and as the shear stress is linearly increased, the shear strain rate increases even more rapidly, producing more flow.
Chemical Properties
For any material, many electrochemical corrosion processes also may occur. Electrochemical corrosion involves two coupled chemical reactions (half cells) at separate sites, connected by two paths. One path (a circuit) is capable of transporting electrons, whereas the other path (an electrolyte) is capable of transferring metallic ions.38 The basic components required for any electrochemical cell are (1) an anode (site of corrosion), (2) a cathode, (3) a circuit, and (4) an electrolyte (Online Fig. 18-14).
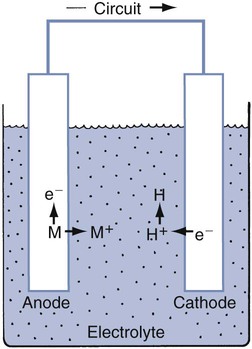
Electrochemical corrosion occurs intraorally when these four components are present. The conditions define which of the metallic sites acts as an anode. Many types of electrochemical cells are possible. Examples are shown schematically in Online Figure 18-15. Many of these electrochemical cells are possible in a single restorative dentistry situation. When an amalgam is in contact with a gold alloy restoration, galvanic, local galvanic, crevice, and stress corrosion are possible. Galvanic corrosion is associated with the presence of macroscopically different electrode sites (amalgam and gold alloy). Local galvanic corrosion (structure-selective corrosion) is caused by the electrochemical differences of different phases in a single material (e.g., amalgam). Electrochemical cells may arise whenever a portion of the amalgam is covered by plaque or soft tissue. The covered area has a locally lowered oxygen or increased hydrogen ion concentration, making it behave more like an anode and corrode (concentration cell corrosion). Cracks and crevices produce similar conditions and encourage concentration cell corrosion. Both corrosion processes are commonly termed crevice corrosion. When the restoration is under stress, the distribution of mechanical energy is not uniform, and this produces different corrosion potentials. This process is called stress corrosion.
Biologic Properties
Finally, toxicology is undergoing rapid evolution. In the 1970s, most toxicologic screening involved the use of the Ames test for determining mutagenicity. The inventor of that test has now withdrawn support for the conclusions derived from that screening procedure.39,40 Results from earlier screening tests of biomaterials may need to be reconsidered.
Biomechanics for Restorative Dentistry
Stress Transfer
The normal tooth structure transfers external biting loads through enamel into dentin as compression (Online Fig. 18-16, A). The concentrated external loads are distributed over a large internal volume of the tooth structure, and local stresses are low. During this process, a small amount of dentin deformation may occur, resulting in tooth flexure. These deformations are discussed in more detail in the following section.
A restored tooth tends to transfer stress differently from how an intact tooth does. Any force on the restoration produces compression, tension, or shear along the tooth–restoration interface.41,42 When enamel is no longer continuous, its resistance is much lower. Most restorations are designed to distribute stresses onto sound dentin, rather than onto enamel (see Online Fig. 18-16, B).43 When in dentin, the stresses are resolved in a manner similar to that in a normal tooth. The process of stress transfer to dentin becomes more complicated when the amount of remaining dentin is thin and the restoration must bridge a significant distance to seat onto thicker dentin (see the section on liners and bases).
For an amalgam restoration in a pulpally deep tooth preparation, 1 to 2 mm of underlying dentin or other insulating material is preferred pulpal of the amalgam to provide adequate thermal and mechanical protection of the pulp.20 If the thickness of dentin is still inadequate, the insertion of an insulating liner or base is recommended. Sometimes it may be necessary, however, to ensure that the amalgam restoration is “seated” on sound dentin at three or more widely separated areas at the level of the initial tooth preparation pulpal wall. This seating provides optimal stress transfer. For a nonmetallic restoration, which has better insulating properties than does a metallic one, 0.5 to 1 mm of dentin or liner or base is sufficient for thermal and mechanical protection.
Strain within Tooth Structure (Tooth Flexure)
Teeth are not rigid structures. They undergo deformation (strain) during normal loading.44 Intraoral loads (forces) vary widely and have been reported to range from 10 N to 431 N (1 N = 0.225 lb of force), with a functional load of 70 N considered clinically normal.45 The number of teeth, type of occlusion, and occlusal habits of patients (e.g., bruxism) affect the load per tooth. The amount of strain is roughly proportional to the amount of stress. Because the tooth structure is heterogeneous and asymmetric, however, and its properties change with time, a simple description of the state of stress or amount of strain does not exist. To date, increasing evidence indicates that the amount of strain and its effect on tooth structure may play a crucial role in fatigue.
Tooth flexure has been described as either a lateral bending or an axial bending of a tooth during occlusal loading.46 This flexure produces the maximal strain in the cervical region, and the strain seems to be resolved in tension or compression within local regions, sometimes causing the loss of bonded Class V restorations in preparations with no retention grooves (Online Fig. 18-17). One current hypothesis is that tensile or compressive strains gradually produce microfractures (called abfractions by some authors) in the thinnest region of enamel at the cementoenamel junction (CEJ) (Online Fig. 18-18).47–50 Such fractures predispose enamel to loss when subjected to toothbrush abrasion and chemical erosion. This process may be key in the formation of some Class V defects (Online Figs. 18-19 and 18-20). Additionally, in unbonded or leaking restorations, this flexure of dentin may produce changes in fluid flow and microleakage, leading to sensitivity and pulpal inflammation. Careful documentation of these events is just beginning.
Effects of Aging
As a tooth becomes older, it undergoes changes in structural mass and in the character of the remaining tissue. Older teeth have lost most prismless enamel along the outer surface and may have encountered numerous microfractures in the cervical portions, as just discussed earlier. In response to disease assaults such as caries or other external stimuli, odontoblastic processes may have laid down more peritubular dentin occluding the outer zones of dentinal tubules.51 Peritubular dentin is mostly hydroxyapatite and tends to stiffen dentin. Secondary and reparative dentin also may have been produced, replacing some of the pulp chamber and canals. Other strong evidence suggests that with aging, all type I collagen in the human body becomes more cross-linked.52 It is strongly suspected that this process of cross-linking makes intertubular dentin more brittle. It is logical that the modulus of teeth is observed to increase with aging (50% increase from 20–29 years of age to 40–49 years of age), and that teeth behave in a more brittle fashion.53 This alteration, coupled with microcracks that may have developed with fatigue, may produce large cracks or fractures in the tooth over time. Supporting bone also may undergo property changes with age.54 These changes produce a substrate that may not transfer stress as readily and that no longer may be well matched to the properties of a restorative material that has survived for a long time. The complete implication of these changes is not yet fully understood.
Direct Restorative Biomaterials
Amalgam
Terminology
Amalgam alloy is a silver–tin alloy to which varying amounts of copper (Cu) and small amounts of zinc (Zn) have been added. Low-copper amalgam alloys contain 2% to 5% copper. The earliest successful amalgams were made by combining filings of such alloys with mercury. A typical modern low-copper amalgam alloy may contain 69.4% silver, 26.2% tin, 3.6% copper, and 0.8% zinc (Online Table 18-3). Amalgams made from such low-copper alloy filings are often referred to as conventional amalgams. High-copper amalgam alloys contain 12% to 30% copper, and because of their higher copper content, these alloys display significantly better corrosion resistance than do low-copper amalgams. A typical high-copper amalgam alloy may contain 60% silver, 27% tin, 13% copper, and 0% zinc (see Online Table 18-3). The particles of these alloys that are mixed with mercury may be filings, but they are often small spheres. Amalgam is mixed for use by combining amalgam alloy particles with mercury, vigorously mixing the components (trituration) for a few seconds during the initial reaction, placing the plastic mass into a tooth preparation, compressing the mixture (condensation) to remove the excess mercury-rich phase, and finally carving and finishing the hardening mass.
Online Table 18-3
Composition and Classification of Dental Amalgam Alloy Powders*
Amalgam Alloys | Classification | Particle Type | Ag | Sn | Cu | Zn | Hg | Other |
New True Dentalloy | Low Copper | Lathe-Cut | 70.8 | 25.8 | 2.4 | 1 | 0 | — |
Micro II | Low copper | Lathe-cut | 70.1 | 21 | 8.6 | 0.3 | 0 | — |
Dispersalloy | High copper | Mixed | 69.5 | 17.7 | 11.9 | 0.9 | 0 | — |
Tytin | High copper | Spherical | 59.2 | 27.8 | 13 | 0 | 0 | — |
Sybralloy | High copper | Spherical |
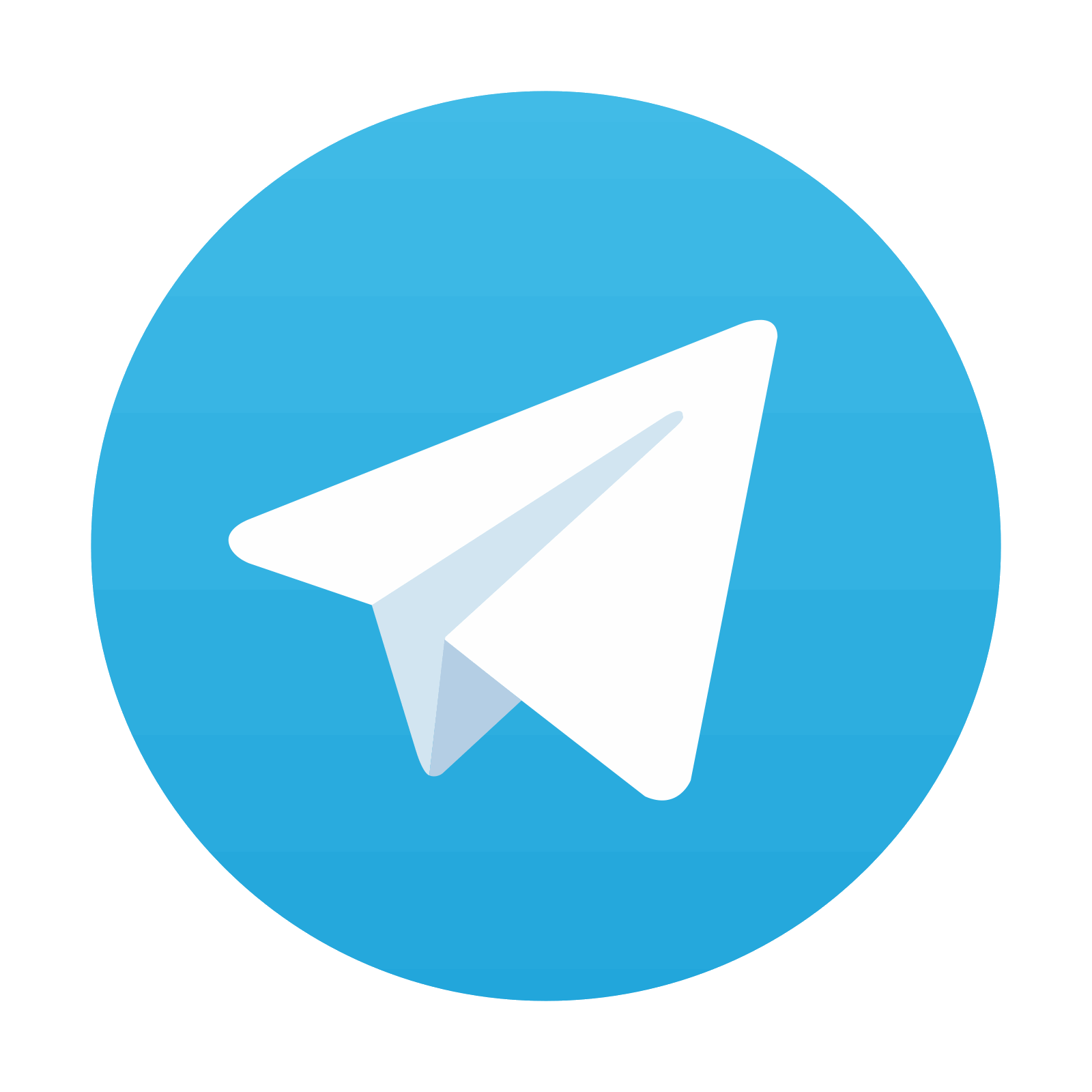
Stay updated, free dental videos. Join our Telegram channel

VIDEdental - Online dental courses
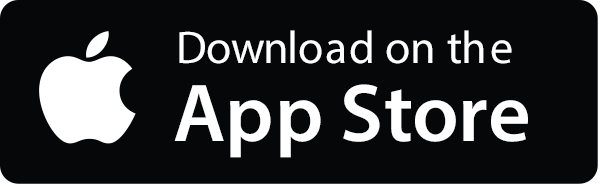
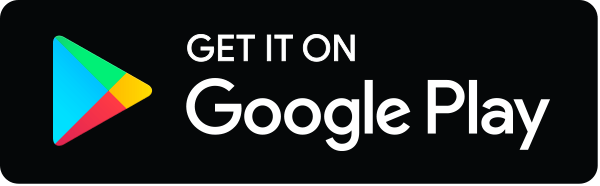