Scientific Rationale for Dental Implant Design
Carl E. Misch, J. Todd Strong, Martha Warren Bidez
The treatment planning sequence for implant dentistry begins with the design of the final restoration.1 After the position and number of teeth replaced and type of prosthesis are determined, the patient force factors are evaluated.2,3 The greater the force factors, the greater the implant support required. The bone density in the region of the implant abutments is then considered, with poorer bone densities requiring a greater amount of implant support.4 The key implant positions and additional implant number are then determined followed by the ideal implant size.5 The available bone in the edentulous sites is then evaluated.6 When the bone available is present for the size, number, and position of the implants for the planned prosthesis, the treatment proceeds with little compromise.
When the available bone is not present, a modification of one or more factors of the treatment plan is necessary. These modifications include (1) bone augmentation to fulfill the ideal treatment plan, (2) consideration of optional implant locations, (3) additional implants, (4) an increase in implant size, and (5) optimization of implant design (Table 15-1). A favorable implant design may help compensate for risk of occlusal loads in excess of normal, poor bone densities, less than ideal implant position or number, or less than an ideal implant size.7
TABLE 15-1
Inadequate Available Bone for Ideal Treatment Plan
Many different implant body designs are available in implant dentistry. They may be categorized as a cylinder type, screw type, press fit, or a combination of features (Figure 15-1). Dental implants are often designed to answer a primary focus or belief that implant failure may stem from (1) implant surgery, (2) bacterial plaque complications, (3) biologic width bone loss, or (4) loading conditions.
Implant Design
Surgical Prospective
In the past, implant body design was driven by the surgical ease of placement. A surgically driven implant design will tend to have a tapered, short implant body or a press-fit insertion. These features permit the implant site preparation and implant to be surgically most easily placed. A cylinder or press-fit implant has a friction-fit insertion and may have less risk of pressure necrosis from too tight an insertion pressure, has no need to bone tap (even in dense bone), and may have the first-stage cover screw already in place because no rotational force is required to insert the implant. As a result, cylinder or press-fit implants are the easiest to insert, were very popular in the 1980s, and were reported to have high initial success rates.8,9 However, after 5 years of loading, reports of the cylinder implant more often include loss of crestal bone and implant failure. For example, in a report by Scortecci et al., the 3-year survival of a press-fit cylinder design with a rough surface condition reported a success rate of almost 100%. However, after 10 years of loading, the implant failure was approximately 50%10 (Figure 15-2). Most likely, this was related to a fatigue overload condition and harmful shear loads on the bone, causing large bone turnover rates and ultimately less bone–implant contact percent and higher risk of overload failure.
The most predictable aspect of implant dentistry appears to be the surgical success. After many years of clinical studies and evaluations, the surgical success rate from implant insertion to uncovery of the implant is usually higher than 98% regardless of the implant design or size.8,9,11–14 As such, designing an implant for surgical ease does not appear to be the most important aspect of the long-term overall implant-prosthetic–related process to reduce the incidence of complications.
Crest Module Considerations
An implant body may be divided into a crest module, the body, and the apex. The crest module of an implant body is the transosteal region, which extends from the implant body and often incorporates the antirotation components of the abutment implant connection (Figure 15-3). The crest module of the implant has a surgical influence, a bacterial plaque concern if bone loss occurs, biological width influence, a loading profile consideration (characterized as a region of highly concentrated mechanical stress), and a prosthetic influence. Therefore, this area of the implant body is a major determinant for the overall implant body design.
Surgical Aspects
During the surgical phase, the crest module design primarily benefits the crestal implant interface. The crest module of an implant should be slightly larger than the outer thread diameter of the implant body. In this way, the crest module seals completely the osteotomy, providing a barrier and deterrent for the ingress of bacteria or fibrous tissue during initial healing. The seal created by the larger crest module also provides for greater initial stability of the implant after placement, especially in softer unprepared bone, because it compresses the crestal bone region.15
“Biologic Width” Designs
There are at least six causes of marginal bone loss at the crestal bone region of implants, including the formation of a “biological width” and occlusal overload after the implant is in function.16,17 The biological width of an implant is related to marginal bone loss before, or separate from, occlusal loading influences. Both of these entities have increased bone loss risk when smooth metal is placed below the bone.
In an animal study, Hermann et al. placed one-piece implants with a 1.5-mm smooth crest module below the bone and compared it with a roughened crest module of similar design level at the bone crest in dogs18 (Figure 15-4). The two implant designs were followed for 6 months, with no occlusal load. Within 1 month after the implant insertion and extension through the soft tissue, marginal bone loss occurred on the smooth region 1.5 mm below the crestal region. No bone loss occurred when the implant crest module was rough and placed at the level of the crestal bone (Figure 15-5). Hammerle et al. inserted similar implant designs in human patients and found a similar crestal relationship to smooth metal below bone.19 Hanggi et al. also compared two different crest module designs in patients and found the longer smooth metal collar had more crestal bone loss.20 The “biologic width” of initial bone loss appears to be directly related to the amount of smooth metal placed below the bone. Therefore, the crest module of the implant should not have an extended smooth metal area placed below the bone.
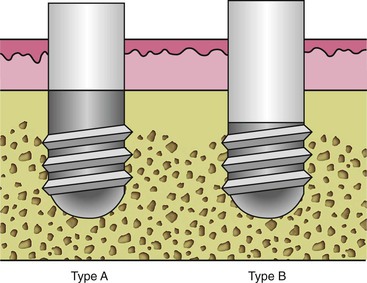
A “biological width” of 0.5 mm has been reported apical to the abutment-to-implant connection in most implant crest module designs, whether or not the crest module is rough. A 0.5-mm-length smooth collar may provide for a desirable smooth surface close to the perigingival area while preserving the biomechanical performance of the remaining portion of the crest module (Figure 15-6). The “microgap” between the implant crest module and abutment may also be eliminated when polished surfaces are approximated.
The soft tissues surrounding a dental implant in several ways are similar to a natural tooth. Both entities have a junctional epithelial “attachment” zone below the sulcus region. The actual “attachment” to the tooth or implant is a hemidesmosome with a layer of glycoaminoglycin. It is not a true attachment because plaque may destroy it, and a dental probe may penetrate through the region. However, in health, it can prevent bacteria in the sulcus from invading the underlying tissues. Below this region on a natural tooth is a connective tissue (CT) attachment zone. The CT attachment is a physical barrier because Sharpey’s fibers of collagen insert into the cementum of the tooth. An implant does not typically have this zone of attachment (Figure 15-7).
Smooth implant surfaces on an implant crest module encourage fibroblast growth and spreading of the cells over the surface, which encourage a fibrous capsule that restricts bone formation. On the other hand, ridge-groove widths similar to the size of cells may guide cell migration and orientation.21 It has been observed from in vitro studies that an 8- and 12-micron microgrooved architecture affects cellular attachment, spreading, orientation, and growth of the fibroblasts and osteoblast cell types. An 8-micron groove was the most effective to inhibit cell migration across the groove, in effect acting as a migration barrier. The 12-micron groove was found to inhibit fibrous tissue growth and permit bone cell growth.
Animal and human histology has observed collagen connection of the soft tissue to microchannels that have three-dimensional structure.22,23 This soft tissue “attachment” zone acts to restrict apical migration of gingival epithelial cells and fibroblasts in the smaller grooves and permit bone tissue to establish attachment to the larger microtextured surface. As a consequence of these studies, three-dimensional microchannels of 8 and 12 microns have been introduced on the crest module (or implant abutment) of an implant(Laser-Lok, BioHorizons Dental Implants) and permit a physical attachment of CT fibers above the bone to the implant surface (Figure 15-8). These three-dimensional microstructures are formed by a computer-controlled laser ablation technique (Figure 15-9). An advantage of the three-dimensional microchannel architecture is that the soft tissue has a base of connection to the implant, similar to a tooth (Figure 15-10). The CT around the implant may physically attach to the implant surface (Figure 15-11). Hence, the soft tissue drape, including the interimplant papilla, may be more stable and improve the soft tissue esthetic regions around an implant prosthesis. This may be particularly advantageous when implants are adjacent to each other and the interimplant papilla is in the esthetic zone (Figure 15-12).
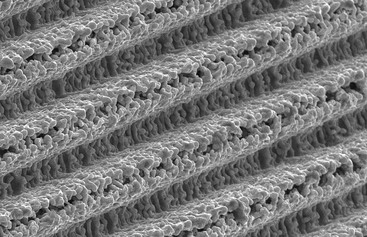
Occlusal Loading Design.
The next consideration of the crest module is related to occlusal loading.17 Most of the occlusal stress occurs at the crestal region of an implant design.16,24 A larger crest module diameter compared with the implant body increases surface area, which can further decrease stress at the crestal region. A crest module height of 2 mm, which is 4.2 mm in diameter compared with a 3.7 mm diameter, has 9% greater surface area (π × Diameter × Height).5 Because the stresses are highest in this region, the greater surface area decreases stress to the bone. In addition, the increase in the diameter of the crest module also affects the strength of the implant body. The crest module diameter increases the strength of the implant more than the surface area because the bending fracture resistance is related to the radius of the crest module times the power of 4.7
In addition to the “biologic width” bone loss before occlusal loading, the cylinder smooth metal reduces the bone cell contact compared with a roughened surface after loading.25 When the surface condition of the implant is rough, the bone–implant contact (BIC) is increased during initial healing. The stress applied to an implant interface with bone may be related to the BIC because stress equals force divided by the area of contact. Reverse torque tests after initial healing observe consistently higher values when roughened surfaces are compared with machined or polished surface conditions.25 Significant loss of crestal bone after loading has been reported for implants with machined (smooth) coronal regions, and the amount of the bone loss is directly related to the length of the smooth crest module.26
Cylinder crest module (or implant) designs promote shear stresses in the adjacent bone interface after loading, regardless of the surface condition27 (Figure 15-13). Bone is 65% weaker to shear loads compared with compression loads and 35% weaker compared with tensile loads28 (Figure 15-14). Hence, a crest module design to limit shear loads is in order. A roughened surface, but a parallel-sided crest module, maintains the bone through the biological-width bone healing period. However, it often loses the marginal bone during the occlusal load conditions because it loads the bone in shear.
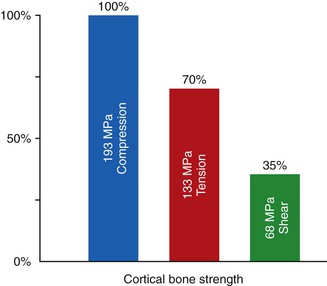
The concept of rough surface condition and shear loads are two different entities, which may act together. Although more bone contact during initial healing is most often evident to the cylinder design rough surface, the stress to the bone after loading is still a shear force. For example, Straumann ITI has a roughened crest module, which is a cylinder below the bone. The initial bone loss before loading is minimal. However, a clinical report on human patients demonstrated almost 30% of the implants lost more than 5 mm of bone. and 22% lost more than 6 mm during a 10-year prospective study29 (Table 15-2). DeBruyn et al. followed clinical outcomes of implants with an internal hex and long rough-cylinder crest module).30 Crestal bone loss was consistently found at least to the first thread, which accounted for more than 2 mm of bone loss. In other words, a rough crest module with a cylinder design may not be sufficient to stop crestal bone loss after the implant is loaded.
TABLE 15-2
Success Rates Using 5 mm as Borderline Compared with Clinical Survival
Implant Type (Clinical Survival) | Number of Implants Placed | <5 mm Bone Loss |
Hollow screw (95.4) | 112 | 101 (74%) |
Hollow cylinder (85.7%) | 9 | 7 (63%) |
Angulated hollow cylinder (91.7%) | 18 | 6 (61%) |
All implants (92.4%) | 179 | 154 (69.8%) |
Data from Karoussis IK, Bragger U, Salvi GE, et al: Effect of implant design on survival and success rates of titanium oral implants: a 10-year prospective cohort study of the ITI Dental Implant System, Clin Oral Implants Res 15:8–17, 2004.
It has been a common clinical observation that bone is often lost to the first thread after loading, regardless of the manufacturer type or design. Bone grows above the threads during initial healing, but after prosthesis loading, bone loss is often observed to this landmark. However, the amount of crestal bone loss is different for different implant designs and related to the length of the crest module. For example, the first thread is 1.2 mm below the platform of the Nobel Biocare Mark IV implant, 2 mm below the platform on the Nobel Replace design, and 3.5 mm on internal hex Zimmer implant designs. The magnitude of the crestal bone loss is often directly related to the distance between the crest module and the first thread distance (regardless of whether the surface condition is smooth or rough above the thread) (Figure 15-15). Therefore, the bone loss cannot be related to a specific “biological width” but may be in part caused by crest module design. The bone loss often stops at the first thread because the first thread changes the shear load created by the cylinder crest module to a component of compressive loading (and the bone is 65% stronger). Instead of designing the crest module for shear, an improved design that loads the bone in compression can reduce the crestal bone loss risk after occlusal loading.
Prosthetic Attachment Designs.
The original external hex design of Brånemark Nobel Pharma used the hex of the implant crest module only during surgery to engage an insertion tool, and allowed the implant to be threaded into the bone.12 The prosthetic components did not engage the hex. Instead, they only rested on the outer periphery of the crest module (Figure 15-16). These original designs were advantageous when the implants were splinted together for complete arch prosthesis because the implant bodies did not have to be completely parallel to each other. However, after implants were used for single tooth replacement or when angled abutment were developed to allow abutments to be more parallel to each other (circa 1986), the hex components of the crest module were engaged by the abutment and fixated together with an abutment screw.
As a general rule, the abutment attaches to an implant body with an internal extension (usually a hex or trilobe design) or an external dimension (i.e., external hex) (Figure 15-17). After the attachment and implant are fixated together with an abutment screw, the overall crest module, abutment, and connections are similar regardless if they are in external or internal hex (Figure 15-18). Therefore, the restoration results relative to esthetics, retention, and so on are similar. However, the consequences of the connection do affect the implant crest module design.
In most internal hex implant designs, the antirotational feature of the abutment is designed within the crest module. As a result, the implant is lower in profile when placed at the crest of the ridge and easier to cover with soft tissue during surgery. In addition, the antirotational feature is often deeper within the implant body compared with the height of external hex implants. The deeper (or higher) the hex profile, the less force is applied to the attachment screw during loading. Hence, abutment screw loosening is reduced when the hex is deeper. It should be noted that if the external hex height was as tall as the internal hex depth, the force on the abutment screw would be similar.
The larger in diameter the crest module, the larger the diameter of the platform dimension of the abutment connection. There is a corresponding stress reduction to the abutment screw during lateral loading related to the platform dimension. In fact, the platform width dimension is more critical to the stress applied to the abutment screw than is the height (or depth) of the antirotational hex of the implant body connection.31
The internal antirotation feature of an implant body is wider than an abutment screw; therefore, the body wall dimension at the crest module is reduced compared with an external hex design. As a result, the threads on the outside of the implant body often cannot be designed at or above the antirotational feature of the internal hex implants. Therefore, internal hex implant bodies more often have cylinder crest module designs, and shear forces are observed above the first implant body thread. The threads on the implant body may progress more crestally with the external hex implant design because the abutment screw diameter is narrower than an internal hex dimension, and the outer body wall of the implant is thicker. Therefore, the thread depth may approach farther toward the crestal region of the implant (see Figure 15-17).
In summary, a crest module design that incorporates an angled geometry or grooves to the crest module, coupled with a surface texture that increases bone contact, will impose a more compressive component to the contiguous bone and decrease the risk of bone loss after loading. By way of example, implants designed with stress transfer features (i.e., Astra, BioHorizons) often exhibit less bone loss after loading in clinical reports14 (Figure 15-19).
Plaque-Related Crest Module Designs.
A focus of several implant designs is to reduce the plaque-related complications of treatment. Implant plaque accumulation is less on smooth metal than rough metal, similar to less plaque adherence on enamel than a rough tooth root.32 With this concept in mind, many crest module designs feature smooth metal surfaces (Figure 15-20). A smooth crest module of the implant is easier to clean related to oral hygiene methods and collects less plaque than rougher surfaces. Therefore, the rationale is that if bone loss occurs at the marginal regions of the implant, the smooth implant surface will harbor less plaque and be easier to clean.
The crest module is initially placed below the bone in most two-piece implant designs. Therefore, the need for daily hygiene and plaque control is not relevant to the crest module unless crestal bone loss occurs. The initial sulcular soft tissue thickness approximates 3 mm above the bone. A toothbrush bristle only extends 0.5 to 1 mm into the sulcus during oral hygiene procedures. Therefore, unless the tissue recedes, even when bone loss occurs on the implant crest module, the gingival sulcus depth prevents access by hygiene procedures to the crest module after crestal bone loss, especially because the gingival sulcus usually increases in depth after marginal bone loss.
The problem with the reduced plaque philosophy is that the smooth crest module is often initially placed below the crest of the bone and is a design that encourages marginal bone loss from the extension of a biological width after implant uncovery and from shear forces after occlusal loading.16,17 As a result, this plaque-reducing design feature increases the periimplant sulcus depth. After the sulcus or pocket is greater than 5 mm, the incidence of anaerobic bacteria increases. Paradoxically, the feature designed to decrease bacteria complications actually increases the risks. In addition, the increase in pocket depth may also result in shrinkage of the tissue and may even expose the implant crest module. This complication then causes poor esthetics of the soft tissue drape.
Implant Body Design
Most implant body complications in the literature are related to early implant failure after loading and marginal bone loss after the loading of the implant–bone interface. Implant failures are most often observed as early loading failures in softer bone types or shorter implant lengths. A review of the literature by Goodacre et al. between 1981 and 2003 found that, after the implant was loaded, failure rates increased to an average of 16% in soft bone.33 Misch reviewed the literature between 1981 and 2004 and found an overall 18% failure rate in implants shorter than 10 mm.34 For example, in a multicenter study of 6 years, Weng et al. reported implant initial healing survival higher than 98%, but 7-mm implants failed 25% of the time within 18 months of loading, and short implants in the posterior maxilla had the highest early loading failure of any intraoral region.35 Therefore, implant body designs should attempt to primarily address the primary causes of complications (i.e., the factors that address the loading conditions of the implant after the implants are placed in function).
Many dentists have stated that “all implants integrate and act the same.” In this concept, the cost of an implant is therefore a major determinant to the selection of an implant. Although almost all implants integrate after initial surgical healing, the bone loss after loading is not similar for all designs. In fact, different implant survival rates and different marginal bone loss after loading have been reported for different implant body designs. For example, a report by Zechner et al. evaluated the periimplant bone over a 3- to 7-year period around functionally loaded, screw-type implants with a machined surfaced V-thread and a sandblasted, acid-etched, square-thread design.36 Both of these implant types had a similar crest module and external hex connection, and all implants were inserted into the anterior mandible (Figure 15-21, A). The range of bone loss in the study was 0.1 to 8.5 mm for the machined V-thread and 0.2 to 4.8 mm for the rough-surface, square-threaded implant. Almost one third of the machined, V-threaded implants lost more than 4 mm of bone compared with fewer than 10% of the square-threaded rough implants. Bone loss of less than 1 mm was reported for almost half of the rough-surface implants compared with only 5% of the machined-surface implants (Figure 15-21, B). The range of bone loss and the incidence of bone loss both indicate implant design and surface condition made a difference in bone loss.
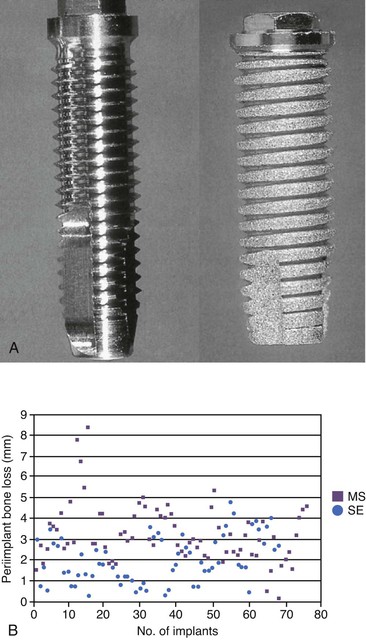
A prospective report by Karoussis et al. also indicated that different implant designs yield different incidences of survival and crestal bone loss.29 Three different implant designs from the same manufacturer (Straumann ITI Dental Implant Systems, Basel, Switzerland) were evaluated with a prospective study over 10 years (see Table 15-2). The survival rate of each design over this time was 95.4% versus 85.7% versus 91.2%. Whereas the implant with the highest survival rate (hollow screw design) lost more than 5 mm of bone in 26% of the implants, the two other designs reported 37% and 39% incidence of greater than 5 mm bone loss. More than 6 mm of marginal bone loss occurred in 22% of implants with the first design and in 35% and 33% for the other two designs.
In summary, several reports indicate implant survival and marginal bone loss after loading were related to implant design. The design of the implant does not affect the surgical healing period (when two-stage implant insertion is used). However, different implant designs influence not only the implant survival but also the amount of early crestal bone loss after loading. The crest module and implant body design both may affect clinical success rates for optimum health.
Functional versus Theoretical Surface Area
An implant body–crest module design can affect the amount of bone loss because the bone loss may be related to biomechanical stress.17 Hence, understanding factors that affect the surface area and type of load to the bone is a benefit to reduce the biomechanical-related complications.
The surface area over which the forces are applied is relevant to bone loss or implant survival and is inversely proportional to the stress observed within the implant system (Stress = Force ÷ Surface area). To reduce risk of biomechanical stress, the force that is applied to the system should decrease or the surface area over which the force is dissipated should increase. This concept is supported by the reduced implant survival reports with short implants.
For a given bone volume, implant surface area should be optimized for functional loads. Thus, an important distinction is made between theoretical total surface area and functional surface area of an implant. Because bone is 65% weaker to shear forces and 35% weaker to tensile forces, functional surface area is defined as the area that actively serves to dissipate compressive loads to the implant–bone interface.37 Functional thread surface area, therefore, is that portion of the implant that participates in compressive load transmission under the action of an axial (or near-axial) occlusal load.
Surface Condition versus Design
In contrast to functional surface area is total theoretical surface area, which may include a “passive” area on the implant that does not participate in load transfer, or has a feature so small that bone cannot adapt to load transfer. An implant has a macroscopic body design and a microscopic component to implant design. Both design features (although independent) are relevant for the clinical behavior. The microscopic features are most important during initial implant healing. The macroscopic implant body design is most important during early loading and mature loading periods. For example, plasma spray coatings are often reported to provide up to 600% more total surface area for the potential BIC.38,39 The roughed surface can increase initial BIC during healing. However, the size of each plasma spray particle is less than 8 microns, and the 120-micron bone cell does not receive a transfer of mechanical stress during loading from this feature. As a result, the amount of actual BIC that can be used for compressive loading may be less than 30% of the total theoretical (microscopic) surface area (Figure 15-22).
Smooth or rough surface, cylindrical implants provide ease in surgical placement; however, the bone–implant interface is subject to significantly larger shear conditions. A cylinder crest module or a cylinder implant body has similar types of force transferred to the bone after loading. A cylinder implant body results in essentially a shear load at the implant–bone interface. Bone grows to a cylinder-shaped implant during initial healing. However, this type of body geometry must rely on a microscopic retention system, such as roughening or coating (e.g., acid etch, mechanical etch, or coatings such as titanium plasma spray or hydroxyapatite [HA]) for the initial loading period.40 The integrity of the implant interface during initial loading is therefore dependent on the shear strength of the implant surface-to-bone bond. The quality of the coating (e.g., HA) is absolutely paramount in such applications.41 If the HA is altered from friction during implant surgical insertion, infection, mechanically removed during treatment of periimplantitis or from bone remodeling over years of function, the remaining smooth-sided cylinder is severely compromised for healthy load transfer to the surrounding tissues42 (Figure 15-23).
The surface conditions of an implant may enhance BIC and adhesion qualities to the bone–implant interface at initial healing. However, the surface coatings on cylinders do not permit compressive forces to be effectively transmitted to the bone cells because the microfeatures of the coating are too small for the cells to be loaded in compression.43 Therefore, the surface area–bone contact percentage is greater during initial healing, but the functional surface area over which loads are effectively dissipated during long-term loading to the surrounding bone is most dependent on the macroscopic design of the implant body. For example, Watzek et al. evaluated machined and rough-surface screw-shaped and rough-surface cylinder implants with histologic and histomorphometric analysis after 18 months of occlusal loading in baboons.44 There was a significant difference in BIC, with screw-type implants having higher values in both the maxilla and mandible (Figure 15-24). The rough-surface screw had slightly more BIC than the machined-surface screw. In addition, the trabecular bone pattern was irregular around the cylinder implants, but the bone was organized and perpendicular to the threads around the screw implants (Figure 15-25). Therefore, the bone trabeculae pattern and the higher BIC resulted in a superior support system for threaded implants.
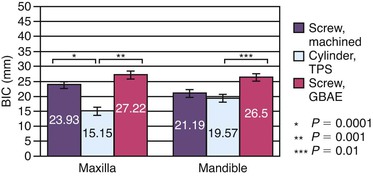
An implant retrieval human clinical report by Bolind et al. evaluated cylinder implants compared with threaded implants from functioning prostheses.45 Consecutively retrieved oral implants from 117 patients, with 85 cylinder implants and 85 threaded implant designs were evaluated. Greater BIC was found around threaded implants, and greater marginal bone loss was observed around cylinder implants (Figure 15-26). It should be noted that whereas the cylinder implants in this report had a roughened surface condition, the threaded implants had a machined surface. Numerous reports demonstrate roughened surfaces have higher BIC compared with machined surfaces during initial healing. In this human retrieval report, therefore, implant body design was more important than the surface condition of the implant for crestal bone loss and overall BIC after loading. In Figure 15-27, an HA-coated cylinder is splinted to two HA-coated threaded implants. After 5 years of loading, more crestal bone loss was observed on the cylinder implant. Hence, implant design may be more important after loading than implant surface condition.
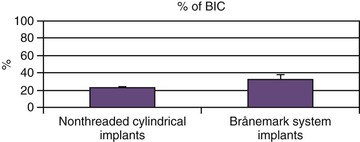
Any shear surface on an implant body increases the risk of bone loss after loading because of inadequate load transfer.25 Figure 15-28 depicts one such example characterized by extensive crestal resorption adjacent to a long, shear surface on the two implant bodies (Core-Vent/Paragon implant). The crestal bone loss contributes to an increase in crown height (which further magnifies stress from bending moments) and the fracture of two abutments. The implant body next to these cylinder-type implants was loaded in the same prosthesis, yet the plateau implant body design (which is a compressive load design) maintained bone height over the years of loading.
Functional Implant Dimension
Another rationale related to functional surface area is related to implant length. An improved functional surface area per unit length of the implant (in contrast to total surface area) is beneficial to reduce the mechanical stress to bone. Most stress to the implant–bone interface in D1 to D2 bone is in the crestal 5 to 9 mm of the implant; therefore, the design of the implant body in the coronal 9 mm is most important to appropriately distribute occlusal stresses to the bone.35,37 For example, a conventional 20-mm V-shaped or reverse buttress–threaded implant of a constant thread depth may have more total surface area compared with a 13-mm square-threaded or plateau design implant. The functional area, however, that is available to resist compressive biomechanical loads in the crestal 5- to 9-mm zone of greatest stress to the BIC may be significantly higher in the 13-mm implant because of the thread geometry.
The diameter of an implant may also affect functional surface area because the length requirements of the implant body are affected by the width of the implant.46 However, the higher bite forces and lower bone density of the posterior regions of the mouth may not always be able to be addressed adequately by only implant diameter. For example, in 1999, Ivanoff et al. found that 6-mm-long implants with a 5-mm diameter had a failure rate of 33% in the mandible and 10% in the maxilla.47 The 8-mm-long, 5-mm-diameter implant yielded 33% and 25% failure rates in the mandible and the maxilla, respectively. On the other hand, the longer 10-mm and 12-mm implants that were 5 mm in diameter yielded no mandibular failures and a 10% failure rate in the maxilla. In other words, the increase in implant diameter alone was not sufficient to improve implant survival. In this report, the 5-mm implant diameter was not enough to compensate for the shorter implants.
Functional Surface Area
Functional surface area is also affected by the variable of initial BIC zones related to bone density upon initial loading. D1 bone, the most dense bone found in the jaws, is also the strongest; has the stiffest modulus of elasticity; and has the highest initial BIC percent, which approximates 80%. D2, D3, and D4 bone have progressively decreasing percentages of bone at the initial implant interface, with D4 bone ranging around 25% interface contact at the initial healing and uncovery of a machined titanium implant.4,48
In addition, the stress transfer to an implant body is affected by the bone density. In D1 and D2 bone with a greater bite force on the implant, the stresses are greatest at the crest and then reduced along the implant body for approximately 5 to 9 mm. In D3 bone, the high crestal stresses with a greater amount of occlusal force may extend farther along the implant body and reduced straining level and extend up to 12 mm of implant length. D4 bone has higher strain conditions and may extend for 12 to 15 mm of implant length, with a high bite force (Figure 15-29).
Therefore, bone density is related to the functional surface area. In more compromised bone sites (i.e., D4 bone), longer implants are required to resist off-axis and moment loads because of cantilevers, improper occlusion, or parafunction.37 Recall that mechanical stress is equivalent to the applied load divided by the surface area over which the load is dissipated. D4 bone has the weakest biomechanical strength and the lowest BIC area to dissipate the load at the implant–bone interface. The functional surface area requirements would increase from a minimum for an implant in D1 bone to a maximum for implants in the D4 bone.7
In summary, bone density, implant diameter, implant length, and implant surface condition may all affect functional surface area; however, the overall implant design may be most effective to decrease biomechanical-related complications. An ideal treatment plan would include an implant length of 12 mm or greater and a 4 mm diameter for most anterior implant sites and 5 mm or greater in the molar regions.5 When the ideal implant size cannot be inserted because of inadequate bone, an alternative to bone augmentation may be to increase the functional surface area of the implant by increasing the implant number or modifying the implant body design.
Bone–Implant Contact Related to Occlusal Load
Different implant survival rates and amounts of marginal bone loss may be directly related to the BIC of different implant body designs. The macrodesign of an implant has an important bearing on the overall surface area to resist the load to the bone. Protruding elements of the implant surface, such as ridges, crests, teeth, ribs, or the edge of threads, may act as stress transfers to the bone when load is applied. Hoshaw et />
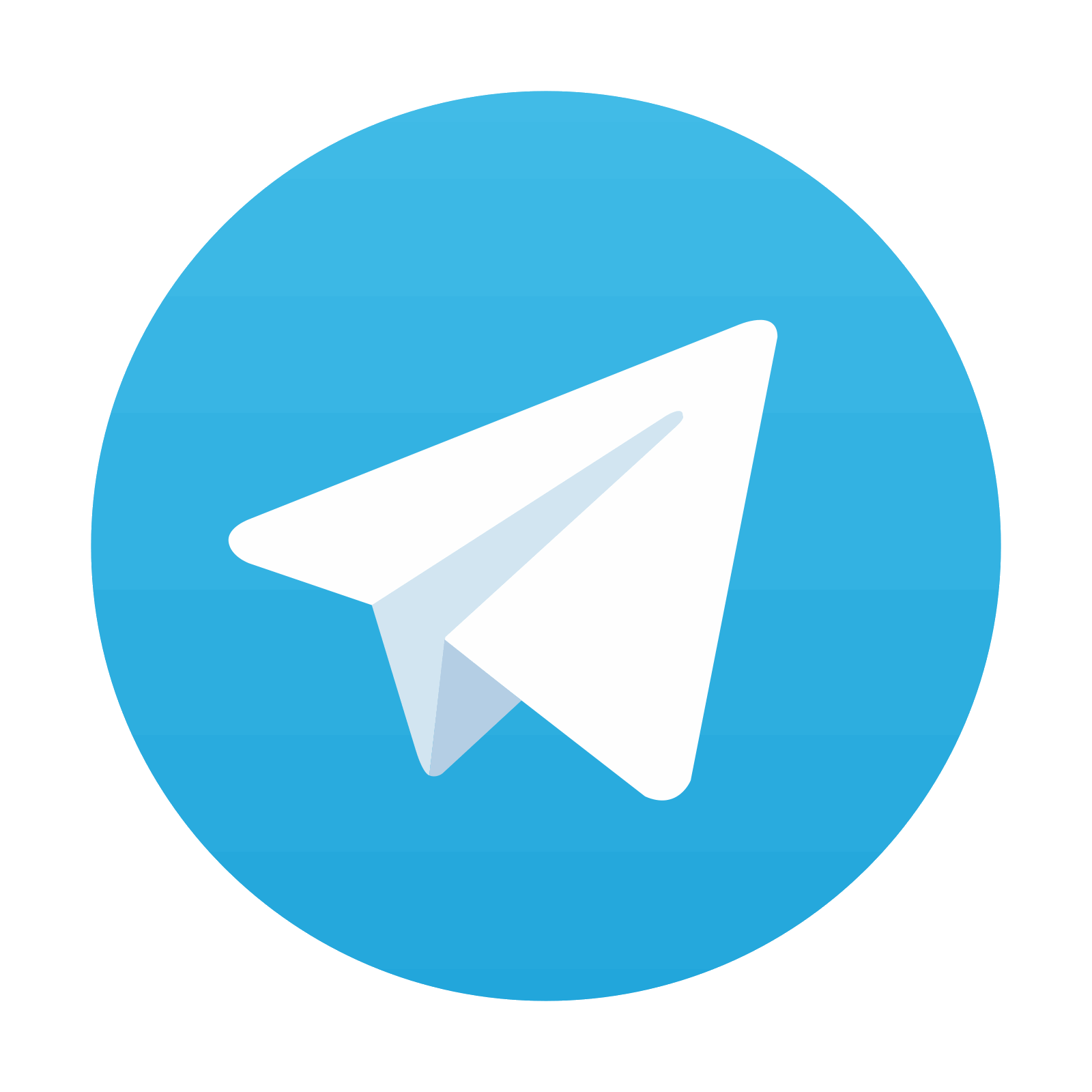
Stay updated, free dental videos. Join our Telegram channel

VIDEdental - Online dental courses
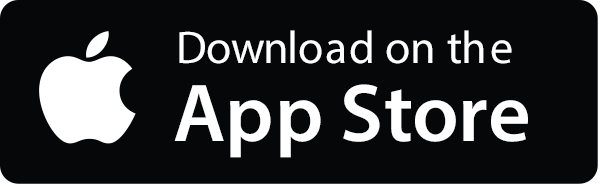
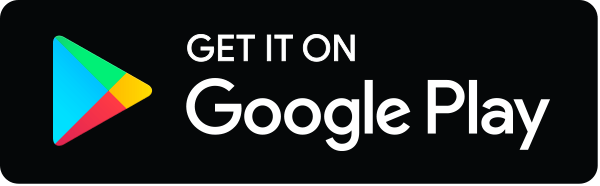