Chapter 24 Biomaterials for Dental Implants
BULK PROPERTIES
History of Materials and Designs
Over the past several decades, definitions of material biocompatibilities have evolved and reflect an ever-changing opinion related to philosophies of surgical implant treatment. In general, the definition of biocompatibility has been given as an appropriate response to a material (biomaterial) within a device (design) for a specific clinical application.1 Metallic and nonmetallic implantable materials have been studied in the field of orthopedics since the turn of the twentieth century.2–7 In the 1960s, emphasis was placed on making the biomaterials more inert and chemically stable within biological environments. The high-purity ceramics of aluminum oxide (Al2O3), carbon, and carbon-silicon compounds and extra-low-interstitial (ELI) grade alloys are classic examples of these trends. In the 1970s, biocompatibility was defined in terms of minimal harm to the host or to the biomaterial. The importance of a stable interaction then moved into central focus for both the research and the clinical communities. In the 1980s the focus transferred to bioactive substrates intended to positively influence tissue responses, whereas in the last two decades, emphasis on chemically and mechanically anisotropic substrates combined with growth (mitogenic) and inductive (morphogenic) substances. Today many biomaterials are being constituted, fabricated, and surface modified to directly influence short- and long-term tissue responses. Bioactive coatings on most classes of biomaterials have continued to evolve from human clinical trials to acceptable modalities of surface preparation, and research focus has shifted to combinations of active synthetic and biological implants.
Of interest, dental implants have significantly influenced these trends. In the 1960s, dental devices were recognized as being in a research and development phase, and critical longitudinal reviews of clinical applications were strongly recommended.8 During this time, longevity studies of various devices demonstrated that the longest duration of clinical applications were for orthopedic prostheses. In the 1980s, controlled clinical trials showed that dental implants provided functional longevities that exceeded most other types of functional tissue replacement modalities.9,10 Clearly, these clinical studies have strongly influenced both the research and development and the clinical application processes. At the present time, the exponential growth of implant use and related scientific reports support the views expressed by early visionaries several decades ago.
Research and Development
Basic studies within the physical and biological sciences have been supportive of the development of surgical implant systems. One example is the continued progress from materials that have been available for industrial applications to the new classes of composites that have evolved for biomedical applications. This same situation exists within a broad area; for example, surface science and technology, mechanics and biomechanics of threedimensional structures, pathways and processes of wound healing along biomaterial interfaces, and the description of the first biofilms that evolve on contact with blood or tissue fluids.11–14 The progressive move from materials to quantitatively characterized biomaterials has been extremely important to the biomedical applications of surgical implants. Dental implant investigations now play a leadership role within selected areas of this overall process, and all phases of medicine and dentistry should benefit.
PHYSICAL, MECHANICAL, AND CHEMICAL REQUIREMENTS FOR IMPLANT MATERIALS
Physical and Mechanical Properties
Forces exerted on the implant material consist of tensile, compressive, and shear components. As for most materials, compressive strengths of implant materials are usually greater than their shear and tensile counterparts. A hypothesis that dental implants are less affected by alternating stresses than implants of the cardiovascular and locomotor systems because of the significantly lower number of loading cycles must be qualified because of the special concern that dental implants are considerably smaller in physical dimension. All fatigue failures obey mechanical laws correlating the dimensions of the material to the mechanical properties of said material.11,15 In addition, when present, parafunction (nocturnal and/or diurnal) can be greatly detrimental to longevity because of the mechanical properties, such as maximum yield strength, fatigue strength, creep deformability, ductility, and fracture. Limitations of the relevance of these properties are mainly caused by the variable shape and surface features of implant designs. A recurring problem exists between the mechanical strength and deformability of the material and the recipient bone. A different approach to match more closely the implanted material and hard tissue properties led to the experimentation of polymeric, carbonitic, and metallic materials of low modulus of elasticity.16,17
Because bone can modify its structure in response to forces exerted on it, implant materials and designs must be designed to account for the increased performance of the musculature and bone in jaws restored with implants. The upper stress limit decreases with an increasing number of loading cycles sometimes reaching the fatigue limit after 106 to 107 loading cycles.11,15,18 In other words, the higher the applied load, the higher the mechanical stress—and therefore the greater the possibility for exceeding the fatigue endurance limit of the material.
In general, the fatigue limit of metallic implant materials reaches approximately 50% of their ultimate tensile strength.11,18 However, this relationship is only applicable to metallic systems, and polymeric systems have no lower limit in terms of endurance fatigue strength. Ceramic materials are weak under shear forces because of the combination of fracture strength and no ductility, which can lead to brittle fracture. Metals can be heated for varying periods to influence properties, modified by the addition of alloying elements or altered by mechanical processing such as drawing, swagging, or forging, followed by age or dispersion hardening, until the strength and ductility of the processed material are optimized for the intended application.
The modifying elements in metallic systems may be metals or nonmetals. A general rule is that constitution or mechanical process hardening procedures result in an increased strength but also invariably correspond to a loss of ductility. This is especially relevant for dental implants. Most all consensus standards for metals (American Society for Testing and Material [ASTM], International Standardization Organization [ISO], American Dental Association [ADA]) require a minimum of 8% ductility to minimize brittle fractures. Mixed microstructural phase hardening of austenitic materials with nitrogen (e.g., stainless steels) and the increasing purity of the alloys seem most indicated to achieve maximum strength and maintain this high level of possible plastic deformation.1,15,19–23
Corrosion and Biodegradation
Corrosion is a special concern for metallic materials in dental implantology, because implants protrude into the oral cavity where electrolyte and oxygen compositions differ from that of tissue fluids. In addition, the pH can vary significantly in areas below plaque and within the oral cavity. This increases the range of pH that implants are exposed to in the oral cavity compared with specific sites in tissue.24–29
Plenk and Zitter15 state that galvanic corrosion (GC) could be greater for dental implants than for orthopedic implants. Galvanic processes depend on the passivity of oxide layers, which are characterized by a minimal dissolution rate and high regenerative power for metals such as titanium. The passive layer is only a few nanometers thick and usually composed of oxides or hydroxides of the metallic elements that have greatest affinity for oxygen. In reactive group metals such as titanium, niobium, zirconium, tantalum, and related alloys, the base materials determine the properties of the passive layer. The stability zones of the oxides of passivable elements cover the redox potentials and pH values typical of the oral environment. However, titanium, tantalum, and niobium oxides cover a markedly larger zone of environmental stability compared with chromium oxides.
The risk of mechanical degradation, such as scratching or fretting of implanted materials, combined with corrosion and release into bone and remote organs has been previously considered. For example, investigators such as Laing,30 Willert et al.,31 and Lemons,32,33 have extensively studied the corrosion of metallic implants. Steinemann34 and Fontana and Greene35 have presented many of the basic relationships specific to implant corrosion. Mears26 addressed concerns about GC and studied the local tissue response to stainless steel and cobalt-chromium-molybdenum (Co-Cr-Mo) and showed the release of metal ions in the tissues. Williams36 suggested that three types of corrosion were most relevant to dental implants: (1) stress corrosion cracking (SCC), (2) GC, and (3) fretting corrosion (FC).
Stress Corrosion Cracking
The combination of high magnitudes of applied mechanical stress plus simultaneous exposure to a corrosive environment can result in the failure of metallic materials by cracking, where neither condition alone would cause the failure. Williams36 presented this phenomenon of SCC in multicomponent orthopedic implants. Others hypothesized that it may be responsible for some implant failures in view of high concentrations of forces in the area of the abutment-implant body interface.37–39 Most traditional implant body designs under three-dimensional finite element stress analysis show a concentration of stresses at the crest of the bone support and cervical third of the implant. This tends to support potential SCC at the implant interface area (i.e., a transition zone for altered chemical and mechanical environmental conditions). This has also been described in terms of corrosion fatigue (i.e., cyclical load cycle failures accelerated by locally aggressive medium). In addition, nonpassive prosthetic superstructures may incorporate permanent stress, which strongly influences this phenomenon under loaded prostheses37,40,41 (Figure 24-1, A and B).
Galvanic corrosion occurs when two dissimilar metallic materials are in contact and are within an electrolyte resulting in current flowing between the two. The metallic materials with the dissimilar potentials can have their corrosion currents altered, thereby resulting in a greater corrosion rate (Figure 24-1, C). Fretting corrosion occurs when a micromotion and rubbing contact occurs within a corrosive environment (e.g., the perforation of the passive layers and shear-directed loading along adjacent contacting surfaces). The loss of any protective film can result in the acceleration of metallic ion loss. Fretting corrosion has been shown to occur along implant body-abutment-superstructure interfaces.
Normally the passive oxide layers on metallic substrates dissolve at such slower rates that the resultant loss of mass is of no mechanical consequence to the implant. A more critical problem is the irreversible local perforation of the passive layer that chloride ions often cause, which may result in localized pitting corrosion. Such perforations can often be observed for iron-chromium-nickel-molybdenum (Fe-Cr-Ni-Mo) steels that contain an insufficient amount of the alloying elements stabilizing the passive layer (i.e., Cr and Mo) or local regions of implants that are subjected to abnormal environments. Even ceramic oxide materials are not fully degradation resistant. Corrosion-like behavior of ceramic materials can then be compared with the chemical dissolution of the oxides into ions or complex ions of respective metallic oxide substrates. An example of this is the solubility of aluminum oxide as alumina or titanium oxide as titania. This statement is generally valid; however, most metallic oxides and nonmetallic substrates have amorphous-hydroxide inclusive structures, whereas bulk ceramics are mostly crystalline. The corrosion resistance of synthetic polymers, on the other hand, depends not only on their composition and structural form but also on the degree of polymerization. Unlike metallic and ceramic materials, synthetic polymers are not only dissolved but also penetrated by water and substances from biological environments. The resulting degree of alteration depends on the material property conditions for the manufactured component.
Toxicity and Consideration
Toxicity is related to primary biodegradation products (simple and complex cations and anions), particularly those of higher atomic weight metals. Factors to be considered include (1) the amount dissolved by biodegradation per time unit, (2) the amount of material removed by metabolic activity in the same time unit, and (3) the quantities of solid particles and ions deposited in the tissue and any associated transfers to the systemic system. For example, the quantity of elements released from metals during corrosion time (e.g., grams per day) can be calculated by using the following formula15:
where TE = toxic element, TEA = toxic elements in alloy, CBR = corrosion biodegradation, and IS = implant surface.
It is of little importance for the formula whether or not the metallic substrate is exposed because the passive layer is dissolved. The critical issue is that the surface represents the “finished” form of the implant. The formula is also valid for ceramic materials and for substances transferred from synthetic polymers. Therefore it appears that the toxicity is related to the content of the materials’ toxic elements and that they may have a modifying effect on corrosion rate.15
Lemons32 reported on the formation of electrochemical couples as a result of oral implant and restorative procedures and stressed the importance of selecting compatible metals to be placed in direct contact with one another in the oral cavity to avoid the formation of adverse electrochemical couples. The electrochemical behavior of implanted materials has been instrumental in assessing their biocompatibility.42 Zitter and Plenk43 have shown that anodic oxidation and cathodic reduction take place in different spaces but must always balance each other through charge transfer. This has been shown to impair both cell growth and transmission of stimuli from one cell to another. Therefore an anodic corrosion site can be influenced by ion transfer but also by other possibly detrimental oxidation phenomena. Charge transfer appears to be a significant factor specific to the biocompatibility of metallic biomaterials. Passive layers along the surfaces of titanium, niobium, zirconium, and tantalum increase resistance to change transfer processes by isolating the substrate from the electrolyte, in addition to providing a higher resistance to ion transfers. On the other hand, metals based on iron, nickel, or cobalt are not as resistant to transfers through the oxidelike passive surface zones.
METALS AND ALLOYS
Several organizations have provided guidelines for the standardization of implant materials.44 ASTM Committee F4 (ASTM F4) and ISO (ISOTC 106, ISOTR 10541) have provided the basis for such standards.19,20 To date, a multinational survey by ISO indicated that titanium and its alloy are mainly used. The most widely used nonmetallic implants are oxidic, carbonitic, or graphitic oxidelike materials.45
Titanium and Titanium–6 Aluminum–4 Vanadium (Ti-6Al-4V)
This reactive group of metals and alloys (with primary elements from reactive group metallic substances) form tenacious oxides in air or oxygenated solutions. Titanium oxidizes (passivates) on contact with room temperature air and normal tissue fluids. This reactivity is favorable for dental implant devices. In the absence of interfacial motion or adverse environmental conditions, this passivated (oxidized) surface condition minimizes biocorrosion phenomena. In situations in which the implant would be placed within a closely fitting receptor site in bone, areas scratched or abraded during placement would repassivate in vivo. This characteristic is one important property consideration related to the use of titanium for dental implants.37,46–48 Some reports show that the oxide layer tends to increase in thickness under corrosion testing,48 and that breakdown of this layer is unlikely in aerated solutions.49
Bothe et al.50 studied the reaction of rabbit bone to 54 different implanted metals and alloys and showed that titanium allowed bone growth directly adjacent to the oxide surfaces. Leventhal51 further studied the application of titanium for implantation. Beder et al.,52 Gross et al.,53 Clarke et al.,54 and Brettle55 were able to expand indications of these materials. In all cases, titanium was selected as the material of choice because of its inert and biocompatible nature paired with excellent resistance to corrosion.1,56–60
The general engineering properties of the metals and alloys used for dental implants are summarized in Table 24-1. Titanium shows a relatively low modulus of elasticity and tensile strength when compared with most other alloys. The strength values for the wrought soft and ductile metallurgic condition (normal root forms and plate form implants) are approximately 1.5 times greater than the strength of compact bone. In most designs in which the bulk dimensions and shapes are simple, strength of this magnitude is adequate. Because fatigue strengths are normally 50% weaker or less than the corresponding tensile strengths, implant design criteria are decidedly important. The creation of sharp corners or thin sections must be avoided for regions loaded under tension or shear conditions. The modulus of elasticity of titanium is five times greater than that of compact bone, and this property places emphasis on the importance of design in the proper distribution of mechanical stress transfer. In this regard, surface areas that are loaded in compression have been maximized for some of the newer implant designs. Four grades of unalloyed titanium and titanium alloy are the most popular. Their ultimate strength and endurance limit vary as a function of their composition.
The alloy of titanium most often used is titanium-aluminum-vanadium. The wrought alloy condition is approximately six times stronger than compact bone and thereby affords more opportunities for designs with thinner sections (e.g., plateaus, thin interconnecting regions, implant-to-abutment connection screw housing, irregular scaffolds, porosities). The modulus of elasticity of the alloy is slightly greater than that of titanium, being about 5.6 times that of compact bone. The alloy and the primary element (i.e., titanium) both have titanium oxide (passivated) surfaces. Information has been developed on the oxide thickness, purity, and stability as related to implant biocompatibilities.9,14,19 In general, titanium and alloys of titanium have demonstrated interfaces described as osteointegrated for implants in humans. In addition, surface conditions in which the oxide thickness has varied from hundreds of angstroms of amorphous oxide surface films to 100% titania (titanium dioxide [Tio2] rutile form ceramic) have demonstrated osteointegration.
The possible influences of aluminum and vanadium biodegradation products on local and systemic tissue responses have been reviewed from the perspectives of basic science and clinical applications.61 Extensive literature has been published on the corrosion rate of titanium within local tissue fluids62–64 and the peri-implant accumulation of “black particles.”65 A few adverse effects have been reported.66 Increased titanium concentrations were found in both peri-implant tissues and parenchymal organs,67,68 mainly the lung and much lesser concentrations in the liver, kidney, and spleen.25,66–70 However, alloy compositions were not well defined or controlled. Corrosion and mechanical wear have been suggested as possible causes.48,67,68 Authors who still caution about the applicability of these results to the presently available titanium alloys have developed other alloys using iron, molybdenum, and other elements as primary alloying agents.17 More recently, several new titanium alloys of higher strength have been introduced.33,71
Although many basic science questions remain, clinical applications of these alloys in dental and orthopedic surgical systems have been very positive, especially in light of improved strength, and the titanium alloys have not demonstrated significant numbers of identifiable negative sequelae.19
Electrochemical studies support the selection of conditions in which elemental concentrations would be relatively low in magnitude.11 Electrochemically, titanium and titanium alloy are slightly different in regard to electromotive and galvanic potentials when compared with other electrically conductive dental materials. Results of these electrochemical potentials and how they relate to in vivo responses have been published previously.9,42,63 In general, titanium- and cobalt-based systems are electrochemically similar; however, comparative elements imitating the conditions in an aeration cell revealed that the current flow in titanium and titanium alloys is several orders of magnitude lower than that in Fe-Cr-Ni-Mo steels or Co-Cr alloys.15 Gold-, platinum-, and palladium-based systems have been shown to be noble, and nickel-, iron-, copper, and silver-based systems are significantly different (subject to galvanic coupling and preferential in vivo corrosion).
The emerging techniques to cast titanium and titanium alloys remain limited for dental implant application because of high melting points of the elements and propensity for absorption of oxygen, nitrogen, and hydrogen, which may cause metallic embrittlement. A high vacuum or ultrapure protective gas atmosphere allows the production of castings in titanium and its alloys at different purity levels,72,73 although microstructures and porosity are relatively unfavorable related to fatigue and fracture strengths.9,32 Typical strengths of cast commercially pure (CP) titanium grade 2 and Ti-6Al-4V after heat treatment and annealing can be in the range of those of wrought titanium alloys used for dental implants.74
Cobalt-Chromium-Molybdenum–Based Alloy
The cobalt-based alloys are most often used in an as-cast or cast-and-annealed metallurgic condition. This permits the fabrication of implants as custom designs such as subperiosteal frames. The elemental composition of this alloy includes cobalt, chromium, and molybdenum as the major elements. Cobalt provides the continuous phase for basic properties; secondary phases based on cobalt, chromium, molybdenum, nickel, and carbon provide strength (four times that of compact bone) and surface abrasion resistance (see Table 24-1); chromium provides corrosion resistance through the oxide surface; and molybdenum provides strength and bulk corrosion resistance. All of these elements are critical, as is their concentration, which emphasizes the importance of controlled casting and fabrication technologies. Also included in this alloy are minor concentrations of nickel, manganese, and carbon. Nickel has been identified in biocorrosion products, and carbon must be precisely controlled to maintain mechanical properties such as ductility. Surgical alloys of cobalt are not the same as those used for partial dentures, and substitutions should be avoided.
In general, the as-cast cobalt alloys are the least ductile of the alloy systems used for dental surgical implants, and bending of finished implants should be avoided. Because many of these alloy devices have been fabricated by dental laboratories, all aspects of quality control and analysis for surgical implants must be followed during alloy selection, casting, and finishing. Critical considerations include the chemical analysis, mechanical properties, and surface finish as specified by the ASTM F4 on surgical implants and the ADA.19,21 When properly fabricated, implants from this alloy group have shown to exhibit excellent biocompatibility profiles.
Iron-Chromium-Nickel–Based Alloys
The surgical stainless steel alloys (e.g., 316 low carbon [316L]) have a long history of use for orthopedic and dental implant devices. This alloy, as with titanium systems, is used most often in a wrought and heat-treated metallurgic condition, which results in a high-strength and high-ductility alloy. The ramus blade, ramus frame, stabilizer pins (old), and some mucosal insert systems have been made from the iron-based alloy.
The ASTM F4 specification for surface passivation was first written and applied to the stainless steel alloys.19 In part, this was done to maximize corrosion-biocorrosion resistance. Of the implant alloys, this alloy is most subject to crevice and pitting biocorrosion, and care must be taken to use and retain the passivated (oxide) surface condition. Because this alloy contains nickel as a major element, use in patients allergic or hypersensitive to nickel should be avoided. In addition, if a stainless steel implant is modified before surgery, then recommended procedures call for repassivation to obtain an oxidized (passivated) surface condition to minimize in vivo biodegradation.
The iron-based alloys have galvanic potentials and corrosion characteristics that could result in concerns about galvanic coupling and biocorrosion if interconnected with titanium, cobalt, zirconium, or carbon implant biomaterials.75–77 In some clinical conditions, more than one alloy may be present within the same dental arch of a patient. For example, if a bridge of a noble or a base-metal alloy touches the abutment heads of a stainless steel and titanium implant simultaneously, then an electrical circuit would be formed through the tissues. If used independently, where the alloys are not in contact or not electrically interconnected, then the galvanic couple would not exist, and each device could function independently. As with the other metal and alloy systems discussed, the iron-based alloys have a long history of clinical applications. Long-term device retrievals have demonstrated that, when used properly, the alloy can function without significant in vivo breakdown. Clearly, the mechanical properties and cost characteristics of this alloy offer advantages with respect to clinical applications.
Other Metals and Alloys
Many other metals and alloys have been used for dental implant device fabrication. Early spirals and cages included tantalum, platinum, iridium, gold, palladium, and alloys of these metals. More recently, devices made from zirconium, hafnium, and tungsten have been evaluated.15,78,79 Some significant advantages of these reactive group metals and their alloys have been reported, although large numbers of such devices have not been fabricated in the United States.
Gold, platinum, and palladium are metals of relatively low strength, which places limits on implant design. In addition, cost-per-unit weight and the weight-per-unit volume (density) of the device along the upper arch have been suggested as possible limitations for gold and platinum. These metals, especially gold because of nobility and availability, continue to be used as surgical implant materials. For example, the Bosker endosteal staple design represents use of this alloy system.80
CERAMICS AND CARBON
Ceramics are inorganic, nonmetallic, nonpolymetric materials manufactured by compacting and sintering at elevated temperatures. They can be divided into metallic oxides or other compounds. Oxide ceramics were introduced for surgical implant devices because of their inertness to biodegradation, high strength, physical characteristics such as color and minimal thermal and electrical conductivity, and a wide range of material specific elastic properties.81,82 In many cases, however, the low ductility or inherent brittleness has resulted in limitations. Ceramics have been used in bulk forms and more recently as coatings on metals and alloys.
Aluminum, Titanium, and Zirconium Oxides
High-strength ceramics from aluminum, titanium, and zirconium oxides have been used for root form, endosteal plate form, and pin type of dental implants.83 The overall characteristics of these ceramics are summarized in Table 24-2. The compressive, tensile, and bending strengths exceed the strength of compact bone by three to five times. These properties, combined with high moduli of elasticity, and especially with fatigue and fracture strengths, have resulted in specialized design requirements for these classes of biomaterials.19,84 For example, the fabrication of a subperiosteal device from a high ceramic should not be done because of the custom nature of these devices, the lower fracture resistance, and the relative cost for manufacturing. The aluminum, titanium, and zirconium oxide ceramics have a clear, white, cream, or light-gray color, which is beneficial for applications such as anterior root form devices. Minimal thermal and electrical conductivity, minimal biodegradation, and minimal reactions with bone, soft tissue, and the oral environment are also recognized as beneficial when compared with other types of synthetic biomaterials. In early studies of dental and orthopedic devices in laboratory animals and humans, ceramics have exhibited direct interfaces with bone, similar to an osteointegrated condition with titanium. In addition, characterization of gingival attachment zones along sapphire root form devices in laboratory animal models has demonstrated regions of localized bonding.9,85–89
One series of root form and plate form devices used during the 1970s resulted in intraoral fractures after several years of function.90 The fractures were initiated by fatigue cycling where biomechanical stresses were along regions of localized bending and tensile loading. Although initial testing showed adequate mechanical strengths for these polycrystalline alumina materials,91 the long-term clinical results clearly demonstrated a functional design-related and material-related limitation. This illustrates the need for controlled clinical investigation to relate basic properties to in vivo performance. The established chemical biocompatibilities, improved strength and toughness capabilities of sapphire and zirconia, and the basic property characteristics of high ceramics continue to make them excellent candidates for dental implants.
Bioactive and Biodegradable Ceramics Based on Calcium Phosphates
Bone Augmentation and Replacement
The calcium phosphate (CaPO4) materials (i.e., calcium phosphate ceramics [CPCs]) used in dental reconstructive surgery include a wide range of implant types and thereby a wide range of clinical applications. Early investigations emphasized solid and porous particulates with nominal compositions that were relatively similar to the mineral phase of bone (Ca5[PO4]3OH). Microstructural and chemical properties of these particulates were controlled to provide forms that would remain intact for structural purposes after implantation. The laboratory and clinical results for these particulates were most promising and led to expansions for implant applications, including larger implant shapes (e.g., rods, cones, blocks, H-bars) for structural support under relatively high-magnitude loading conditions.92,93 In addition, the particulate size range for bone replacements was expanded to both smaller and larger sizes for combined applications with organic compounds. Mixtures of particulates with collagen, and subsequently with drugs and active organic compounds such as bone morphogenetic protein (BMP), increased the range of possible applications. Over the past 20 years, these types of products and their uses have continued to expand significantly.93–96
Endosteal and Subperiosteal Implants
The first series of structural forms for dental implants included rods and cones for filling tooth root extraction sites (ridge retainers)97 and, in some cases, load-bearing endosteal implants.98 Limitations in mechanical property characteristics soon resulted in internal reinforcement of the CPC implants through mechanical (central metallic rods) or physicochemical (coating over another substrate) techniques.99,100
The numbers of coatings of metallic surfaces using flame or plasma spraying (or other techniques) increased rapidly for the CPCs.93 The coatings have been applied to a wide range of endosteal and subperiosteal dental implant designs, with an overall intent of improving implant surface biocompatibility profiles and implant longevities (and are addressed later in this chapter).101–103
Advantages and Disadvantages
Box 24-1 summarizes the advantages and disadvantages of CPCs.
The recognized advantages associated with the CPC biomaterials are as follows104:
Some of the possible disadvantages associated with these types of biomaterials are as follows:
Critical to applications are the basic properties of these substances. Table 24-3 provides a summary of some properties of bioactive and biodegradable ceramics. In general, these classes of bioceramics have lower strengths, hardnesses, and moduli of elasticity than the more chemically inert forms previously discussed. Fatigue strengths, especially for porous materials, have imposed limitations with regard to some dental implant designs. In certain instances, these characteristics have been used to provide improved implant conditions (e.g., biodegradation of particulates).
Calcium aluminates, sodium-lithium invert glasses with CaPO4 additions (Bioglass or Ceravital), and glass ceramics (AW glass-ceramic) also provide a wide range of properties and have found extended applications.96,100
Bioactive Ceramic Properties
The atomic relationships of the basic elements, stoichiometric ratios, and the normal chemical names for several characterized CPCs are provided in Table 24-4. The general family of apatites has the following formula:
Very often, apatite atomic ratios are nonstoichiometric; that is, 1 mol of apatite may contain fewer than 10 mol of metallic ions (M2+) and fewer than 2 mol of anions Z−1.105 The number of XO retains a number of 6. Multiple metals and anions can be substituted within this formulation. Most important, the relative physical, mechanical, and chemical properties of each final CaPO4 material, including each of the apatites, are different from one another.89,95 Additionally, the microstructure of any final product (solid structural form or coating) is equally important to the basic properties of the substance alone. The crystalline monolythic hydroxyapatite (HA) (fired ceramic Ca10[PO4]6[OH]2) of high density and purity (50 maximum ppm impurities) has provided one standard for comparison related to implant applications. The ratio of calcium to phosphorus of Ca10(PO4)6(OH)2 is 1.67, and the ceramic can be fully crystalline. Considerable differences exist between the synthetic HA ceramics (HAs) that are produced by elevated temperature processing and biological apatites (HAs).105 Biological apatites contain trace amounts of Co32−, sodium, magnesium, fluorine, and chlorine ions. These exist in varying ratios and distributions and of course are only one phase of calcified tissues.
The crystalline tricalcium phosphate (βCa3[PO4]2) (TCP) ceramic has also provided a high-purity (<50 ppm maximum impurities) biomaterial for comparison with other products. National standard specifications related to the basic properties and characteristics of both HA and TCP have been published.19 These two compositions have been used most extensively as particulates for bone augmentation and replacement, carriers for organic products, and coatings for endosteal and subperiosteal implants.
Forms, Microstructures, and Mechanical Properties
Particulate HA, provided in a nonporous (<5% porosity) form as angular or spherically shaped particles, is an example of a crystalline, high-purity HA biomaterial106 (Figure 24-2, A). These particles can have relatively high compressive strengths (up to 500 MPa), with tensile strengths in the range of 50 to 70 MPa. Usually, dense polycrystalline ceramics consisting of small crystallites exhibit the highest mechanical strength, apart from monocrystalline ceramics free of defects (e.g., single- crystal sapphire implants). Ceramics are brittle materials and exhibit high compressive strengths compared with tensile strengths. However, less resistance to tensile and shear stresses limit their application as dental implants because of mechanical constraints of implant form and volume. Nonresorbable, “bioinert” ceramics exhibiting satisfactory load-bearing capability are limited to dense monocrystalline and polycrystalline aluminum, zirconium, and titanium oxide ceramics. These same mechanical characteristics exist for the solid portions of several porous HA particulates and blocks. The macroporous (>50 μm), or microporous (<50 μm) particulates have an increased surface area per unit volume. This provides more surface area for solution- and cell-mediated resorption under static conditions and a significant reduction in compressive and tensile strengths (Figure 24-2, B and C; Figure 24-3). The porous materials also provide additional regions for tissue ingrowth and integration (mechanical stabilization) and thereby a minimization of interfacial motion and dynamic (wear-associated) interfacial breakdown. The strength characteristics after tissue ingrowth would then become a combination of the ceramic and the investing tissues.107
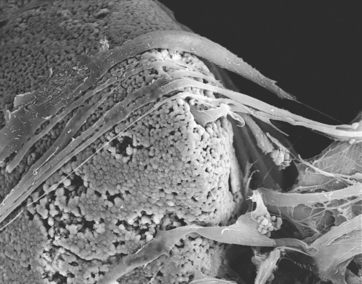
Figure 24-3 Scanning electron microscopy of cells, which actively endocytosed fragments of granules (×1500).
(Courtesy Ceramed Corp, Denver, Colo.)
A number of the CPCs are phase mixtures of HA and TCP, whereas some compounds are composites or mechanical mixtures with other materials93 (see Table 24-4). These classes of bioactive ceramics, including glasses, glass-ceramics, mixtures of ceramics, combinations of metals and ceramics, or polymers and ceramics, exhibit a wide range of properties. In general, these biomaterials have shown acceptable biocompatibility profiles from laboratory and clinical investigations. Bulk-form implant designs made from CPCs, which were contraindicated for some implant designs because of poor mechanical performance, have found a wide range of indications as coatings of stronger implant materials.
The coatings of CPCs onto metallic (cobalt- and titanium-based) biomaterials have become a routine application for dental implants. For the most part these coatings are applied by plasma spraying, have average thickness between 50 and 70 μm, are mixtures of crystalline and amorphous phases, and have variable microstructures (phases and porosities) compared with the solid portions of the particulate forms of HA and TCP biomaterials.93,108 At this time, coating characteristics are relatively consistent, and the quality control and stricter quality assurance programs from the manufacturers have greatly improved the consistency of coated implant systems. (A more detailed discussion of surface treatment options is presented in the next section.)
Concerns continue to exist about the fatigue strengths of the CaPO4 coatings and coating-substrate interfaces under tensile and shear loading conditions. There have been some reports of coating loss as a result of mechanical fracture, although the numbers reported remain small.89 This has caused some clinicians and manufacturers to introduce designs in which the coatings are applied to shapes (geometric designs) that minimize implant interface shear or tensile loading conditions (e.g., porosities, screws, spirals, plateaus, vents). From theoretic considerations, the coating of mechanically protected areas seems most desirable.
Density, Conductivity, and Solubility
Bioactive ceramics are especially interesting for implant dentistry because the inorganic portion of the recipient bone is more likely to grow next to a more chemically similar material. The bioactive (bioreactive) categorization includes CaPO4 materials such as TCP, HA, calcium carbonate (corals), and calcium sulfate–type compounds and ceramics. A chemical-biochemical contact between the host bone and grafted material may be developed, as well as a possible stimulus of bone activity.95 Their limitations have been associated with the material forms that have lower strengths (i.e., similar to or less than bone).95
Dissolution characteristics of bioactive ceramics have been determined for both particulates and coatings.109,110 In general, solubility is greater for TCP than for HA. Each increase relative to increasing surface area per unit volume (porosity) and the CPC solubility profiles depend on the environment (e.g., pH, mechanical motion).
If one considers a uniform material chemistry, then the larger the particle size is, the longer the material will remain at an augmentation site. Thus 75-μm particles will be resorbed more rapidly than 3000-μm particles. In addition, the porosity of the product affects the resorption rate. Tofe et al.111 reported on the porosity of dense, macroporous and microporous CaPO4. Some of the dense HA lacks any macro- or microporosity within the particles. The longest resorption rate occurred with the dense nonporous HA type because osteoclasts may only attack the surface and cannot penetrate the nonporous material. Macroporous CaPO4 (e.g., corallin HA) demonstrated 100-μm or 500-μm pores, which comprised 15% or more of the total material volume. Minimal porosity was found in the HA bulk material that surrounded the large pores. Microporous apatites often have their origin from bovine or human bone. The porosity observed in these materials is approximate/>
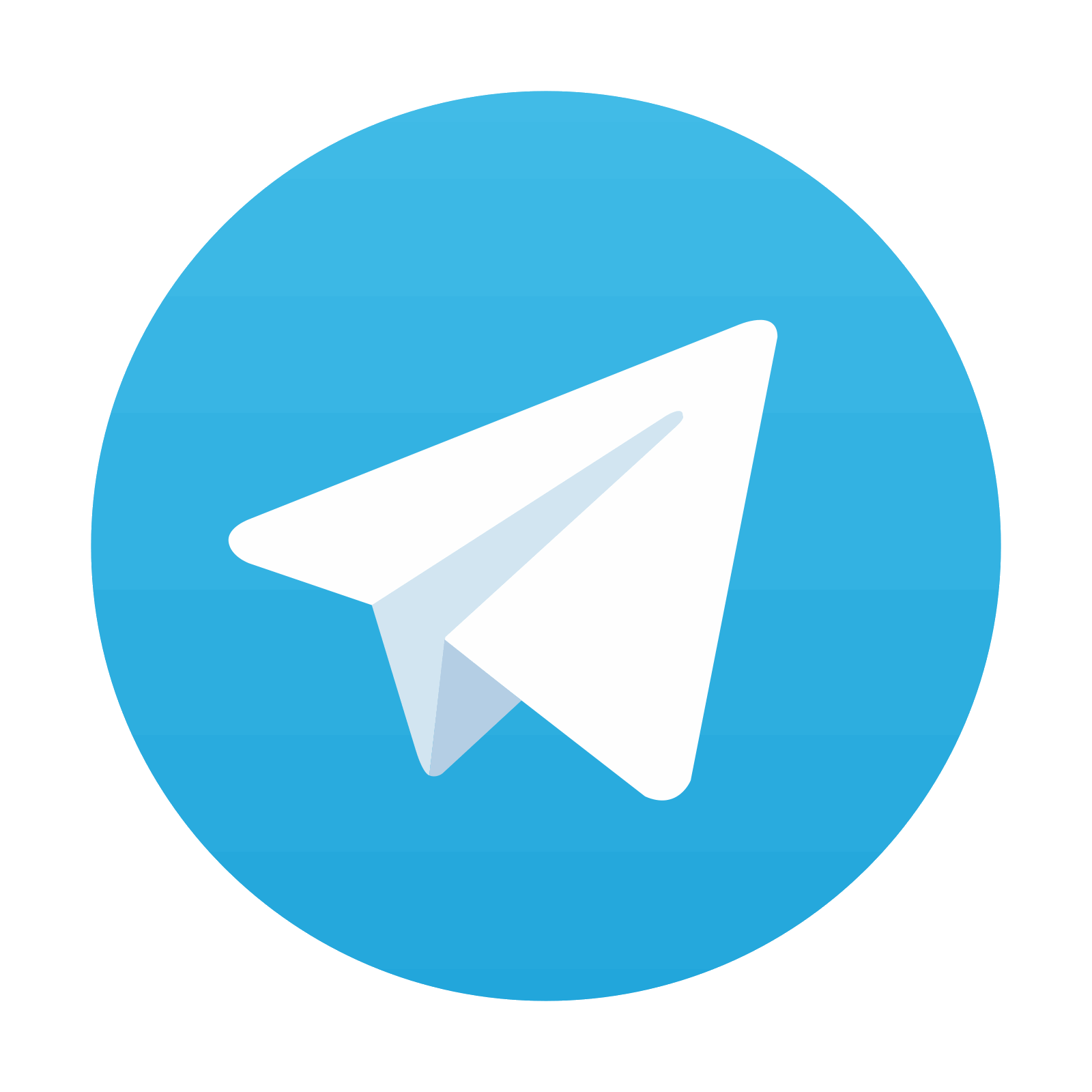
Stay updated, free dental videos. Join our Telegram channel

VIDEdental - Online dental courses
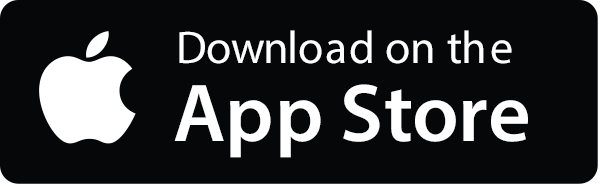
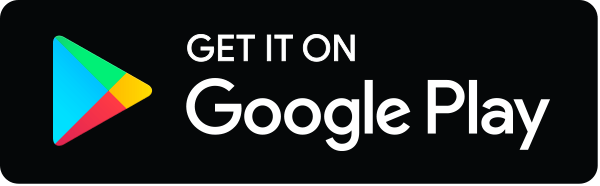