Oral maxillofacial surgeons have a number of approaches for reconstruction of soft and hard tissue defects. Autogenous, allogeneic, alloplastic, and xenogeneic options have been available for years and are continuing to evolve. The current “gold standards” of autogenous soft and hard tissues have inherent donor site morbidity, which limits their use in certain applications. The field of tissue engineering has exploded not as a means of repair but as a common goal of regeneration whereby tissues are re-established into their pre-injury state with minimal to absent native tissue scar formation while attempting to minimize risk and morbidity. Materials and techniques continue to evolve for effective and unique alternatives of repair and are providing a bridge for the regenerative strategies of the future. Current tissue-engineering goals for any application encourage the re-establishment of three-dimensional form and function while using scaffolds, cells, biologic factors, and both biomechanical and biophysical stimulation to assist in regeneration of tissues through manipulation of the host environment. Knowing the mechanical properties of all hard tissues to be re-established and providing unique microenvironments for each tissue type are essential for recapitulation of the intricate form of the cranio-maxillofacial region. The difficulty in this field, in general, is that specific applications in other regions of the body cannot always be extrapolated to the head and neck.
Etiopathogenesis/Causative Factors
A variety of conditions may necessitate either soft or hard tissue reconstruction. There can be a substantial traumatic or ablative defect involving superficial soft tissues and skin. Certain congenital syndromes may also necessitate soft tissue bulk, such as Parry-Romberg syndrome (hemifacial atrophy) and hemifacial microsomia. Hard tissue needs are more wide ranging, depending on the site, and are based on etiology: developmental, traumatic, pathologic, and/or inflammatory. Some wounds prove very difficult to reconstruct because of substantial inflammation, foreign body reaction from previous surgical efforts, scar tissue formation, compromised vascularity, abnormal anatomy, bilateralism, or large composite tissue loss necessitating reconstruction of multiple hard and soft tissue components. An understanding of the specific structural and functional needs of the injured site is imperative before surgery.
Reconstructive Goals
The ultimate goal of regeneration is to provide esthetics, minimize scarring, and maximize function across all fields. To accomplish this task effectively, several principles must be adhered to, and these may vary between reconstruction of hard and soft tissues.
Soft Tissue Principles
- •
Be of appropriate bulk and plasticity
- •
Reconstruct like tissues (skin, mucosa, muscle, fat, fascia, tendon, ligaments, or a combination thereof)
- •
Have unrestricted function
- •
Be reliable with minimal tissue loss during healing
- •
Possess advantageous scarring characteristics
Hard Tissue Principles
- •
Replace complex three-dimensional geometries
- •
Be biocompatible with minimal inflammatory response
- •
Assume load bearing and unrestricted function
- •
Be bioabsorbable to allow transition of load to regenerating structures
- •
Possess mechanical properties similar to those of native tissue
- •
Allow hard tissue ingrowth
- •
Be reliable to avoid mechanical failure
- •
Stimulate site-specific tissue formation to restore function
Reconstructive Goals
The ultimate goal of regeneration is to provide esthetics, minimize scarring, and maximize function across all fields. To accomplish this task effectively, several principles must be adhered to, and these may vary between reconstruction of hard and soft tissues.
Soft Tissue Principles
- •
Be of appropriate bulk and plasticity
- •
Reconstruct like tissues (skin, mucosa, muscle, fat, fascia, tendon, ligaments, or a combination thereof)
- •
Have unrestricted function
- •
Be reliable with minimal tissue loss during healing
- •
Possess advantageous scarring characteristics
Hard Tissue Principles
- •
Replace complex three-dimensional geometries
- •
Be biocompatible with minimal inflammatory response
- •
Assume load bearing and unrestricted function
- •
Be bioabsorbable to allow transition of load to regenerating structures
- •
Possess mechanical properties similar to those of native tissue
- •
Allow hard tissue ingrowth
- •
Be reliable to avoid mechanical failure
- •
Stimulate site-specific tissue formation to restore function
Treatment
The use of scaffolds and constructs is instrumental for regeneration of both soft and hard tissue. Science, to date, has not been able to regenerate complex tissue defects without a framework to build on. These scaffolds can be developed by a variety of means (outlined later) to satisfy site-specific requirements. Although a scaffold itself will also allow minimal promotion of reparative tissues alone, multiple biologic agents (some still yet to be determined) are required to augment the desired tissue response. It is clear that the body possesses complex interdependent interactions during growth, injury, and healing. Manipulation of these interactions could promote the induction of a fetal healing phase that would be ideal to minimize scarring and effectively regenerate the desired tissues.
Modulation of the host environment is desirable to achieve these goals. Inflammation is an innate healing response and is necessary to allow regeneration; however, limiting excessive inflammation is necessary to prevent scar formation, which may be detrimental. It has become clear that a number of inflammatory mediators are important in facilitating a proper healing response, including tumor necrosis factor-α (TNF-α), interleukin-1 (IL-1), and 6 (IL-6). Attempts at decreasing these cytokines with inhibitory agents have been shown to alter the healing response such that the risk for infection is increased and regenerate tissue formation is decreased. More site-specific cytokines continue to be identified, and it is perceivable that their complex modulation (both increase and decrease) may promote appropriate site-specific healing with fewer side effects.
Extensive research has been applied to the evaluation of known biologics, including transplanted cells (undifferentiated stem cells and differentiated progenitor cells), growth factors, and biomechanical and biophysical stimulants ( Fig. 9-1 ). Combining these biologics with basic or complex scaffolds is critical for site-specific soft and hard tissue regeneration. More recently, interest has taken hold in developing an engineered vascular network for blood supply with a composite framework for improved reconstructive options with both hard and soft tissue components.
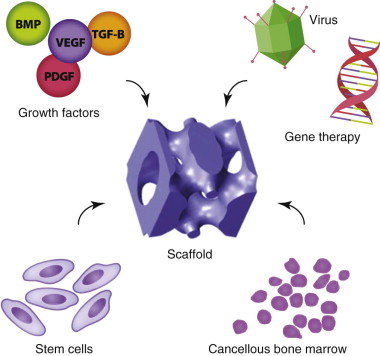
Biologics
Biophysical and Biomechanical Stimulation
Ultrasound and electrical fields offer a means of biophysical stimulation that enhances bone repair. Ultrasound using low-intensity pulsed signals (30 mW/cm 2 ) has shown some benefit in accelerating bone healing during fracture repair and distraction procedures. The results of several studies have demonstrated some benefits in improving function, but they are limited in their overall bone-healing response. Electromagnetic signals can be provided through direct current, capacitive coupling, inductive coupling, or combinations thereof. Use of direct current is limited because of the creation of an inflammatory-type response. Capacitive coupling (electrical fields exclusively) and inductive coupling (electromagnetic fields) alter cytosolic calcium concentrations through voltage-gated calcium channels and intracellular release, respectively. Pulsed electromagnetic fields have been shown to promote osteoblast formation and the production of osteogenic cytokines such as bone morphogenetic proteins (BMP-2 and BMP-4) and transforming growth factor-β (TGF-β). Great interest has been directed toward using this modality with tissue engineering to augment current and future strategies for bone healing.
Promising results have been achieved in nerve repair as well. Future investigation is focusing on the use of implantable devices for stimulation during bone repair and reconstruction.
Distraction Osteogenesis
Distraction was first described by Ilizarov in 1969 and has given us insight into native bone-healing capacity. By providing steady traction or tension on a stable callus, tissues can be augmented in a variety of ways for condylar, alveolar, and segmental reconstruction. Distractor devices have evolved over the years from an external device to more compact internal devices that can be placed intraorally to minimize extraoral scar defects. Further research is being conducted to create smaller bio-expandable devices that possess the appropriate strength and are self-activated by an internal motor mechanism.
Cellular Delivery
With the use of scaffolds, delivery of biologic agents has been of keen interest to researchers. Stem cells and differentiated progenitor cells have their own indications, depending on location and desired regeneration strategy. A great amount of work is still necessary to idealize treatment outcomes in animals even before trials in humans are conducted. Stem cell therapy continues to be on the forefront given the ability to direct differentiation and enhance tissue regeneration through the provision of specific cell lines. Stem cells located throughout the body can be cultured from various tissue sources to stimulate cell-specific differentiation and expansion. Hard tissue regeneration focuses on the pluripotency of bone marrow stromal stem cells (also known as mesenchymal stem cells), hematopoietic stem cells, and adipose stem cells to differentiate into chondrocytes and osteoblasts. Further interest has peaked with harvest and manipulation of dental pulp stem cells, especially those from extracted wisdom and primary teeth ( Fig. 9-2 ). Several for-profit companies have marketed collections of extracted teeth to be made available for future stem cell use:
- •
National Dental Pulp Laboratory, Inc., Newton, Mass
- •
BioEden, Inc., Austin, Texas
- •
StemSave, New York, New York
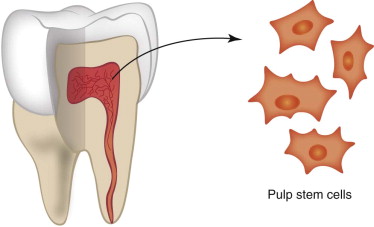
The non-profit National Stem Cell Bank has been relocated to WiCell (Madison, Wisc.) for cGMP (current good manufacturing practices) research cell lines. These cells can be differentiated and expanded ex vivo or relocated and induced within the desired site of reconstruction. Once cells have been delineated, they can be incorporated directly within the fabricated scaffolds by using hydrogels to precisely deliver to the reconstructed area cells that possess characteristics similar to the tissues to be repaired or regenerated.
Growth Factors
More recently, growth factors have been explored for specific applications to manipulate the host environment for promotion of repair and regeneration ( Table 9-1 ). Specific growth factors for head and neck applications include recombinant human bone morphogenetic proteins (rhBMP-2 and rhBMP-7), platelet-derived growth factor (PDGF), vascular endothelial growth factor (VEGF), TGF-β, and insulin-like growth factor type I (IGF-I).
GROWTH FACTOR | SOURCE | EFFECTS |
---|---|---|
BMP | Bone matrix, osteoprogenitor cells (periosteum and ostium), fibroblasts, proliferating chondrocytes | Mesenchymal cells differentiated into osteoblasts, matrix deposition |
PDGF | Platelets, keratinocytes, macrophages | Chemotaxis and activation of macrophages and fibroblasts, matrix deposition; migration and proliferation of osteoblasts, cementoblasts, and periodontal ligament |
VEGF | Keratinocytes, macrophages | Neovascularization and angiogenesis; indirectly involved with osteoprogenitor cell differentiation |
TGF-β | Macrophages, platelets, keratinocytes | Keratinocyte migration, proliferation, remodeling; chondrogenesis |
IGF-I | Plasma, platelets | Endothelial cell and fibroblast proliferation |
FGF | Macrophages, fibroblasts, endothelial cells | Angiogenesis, fibroblast proliferation |
Bone Morphogenetic Proteins
rhBMP-7 (OP-1, Stryker, Portage, Mich.) is currently available only for posterolateral and lateral spinal fusions. The Food and Drug Administration (FDA) has authorized the use of rhBMP-2 (Infuse, Medtronic, Minneapolis, Minn.) for sinus lift and alveolar augmentation procedures, although trials are currently being conducted to identify success in other maxillofacial procedures. BMPs target the undifferentiated mesenchymal stem cell to differentiate into osteoblasts through direct receptor stimulation and signaling.
Platelet-Derived Growth Factor
Ultra-concentrated formulations of PDGF (Gem 21s, Osteohealth, Shirley, NY) have proved beneficial for periodontal defects and small alveolar defects. PDGF has potent chemotactic and mitogenic properties for bone regeneration, with both migration and proliferation of osteoblasts, cementoblasts, and periodontal ligament fibroblasts. In addition, PDGF has been implicated in promoting soft tissue wound healing through chemotaxis of fibroblasts and subsequent stimulation of proliferation and matrix deposition for wound repair. One such product is marketed for the treatment of non-healing ulcers, especially in diabetics (Regranex, Systagenix Wound Management, Quincy, Mass.).
Vascular Endothelial Growth Factor
Neovascularization is up-regulated with VEGF, which is also involved in the differentiation of osteoprogenitor cells through the delivery of nutrients and oxygen to injured areas, as well as secretion of growth factors and cytokines by endothelial cells of the newly developed vessels. The role of VEGF on chondrocytes, osteoclasts, and osteoblasts is well documented, with evolution of endochondral bone formation and improvement of bone mineralization.
Other Growth Factors
Other growth factors such as TGF-β, IGF-I, and basic fibroblast growth factor (bFGF) are useful for cartilage and soft tissue promotion. Multiple isoforms of TGF-β have been isolated and found to contribute to keratinocyte migration, matrix synthesis, and remodeling, as well as chondrogenesis and bone regeneration with minimal scarring at different stages of the healing cascade. Some may additionally be involved in inflammation and cause fibrosis with scarring. It is therefore critical to further elucidate beneficial formulations for improving wound healing. FGF in particular has been shown to be exceptionally useful for soft tissue repair as a result of its promotion of angiogenesis and keratinocyte migration and mitogenesis. Combinations of these growth factors are being evaluated and proving to be beneficial for numerous maxillofacial applications. Further large-scale prospective randomized trials are needed to determine the proper concentration and application of these materials. Such materials should be used cautiously; off-label use is not advocated given their substantial systemic effects and risks.
Gene Therapy
Gene therapy has been investigated by numerous teams for improving delivery and bioavailability of the critical growth factors just listed for both soft tissue and bone regeneration. Concerns regarding high concentrations of vectors, gene over-expression, or disturbance of native cell signaling have limited mainstream acceptance by the FDA, and gene therapy may therefore not be available for routine human applications for years to come. New technologies have evaluated reactive materials to allow spatial control of the delivery of gene vectors without substantial diffusion to outlying tissues. Such substrate site-specific delivery allows the use of decreased levels of viral vectors for local transduction. Any of the aforementioned growth factors can then be delivered directly to the site without worrying about diffusion and can be combined to influence differential tissue regeneration.
Specific Treatment and Techniques
Soft Tissue Reconstruction
Oral Mucosa and Skin
A large amount of research is being conducted to identify strategies for minimizing the scarring and donor site morbidity related to skin and mucosal grafting in the head and neck region to treat post-ablative defects and burn injuries, as well as for pre-prosthetic purposes. A variety of approaches have been used in the past, including split- and full-thickness skin grafts, oral mucosa free grafts, and oral connective tissue grafts. Standard, orally harvested grafts are restricted to the treatment of small defects because of their limited supply and significant discomfort at the donor site. More recently, deepithelialized acellular cadaveric dermal grafts have been used but have demonstrated varied results of healing and scarring in treating lower eyelid, alveolar, and tongue wounds, where they act more as a biologic barrier during the healing process. In addition, because burn victims may have few sites available for graft harvest, cell culture techniques have been investigated for aerosolization and uniform seeding of keratinocytes across the wound bed. Trials are still ongoing to determine effectiveness in humans, but products are currently on the market (CellSpray, Avita Medical Americas, Woburn, Mass.).
A newer method of creating a tissue-engineered soft tissue substitute is expansion of a small number of oral keratinocytes onto an underlying dermal scaffold. A soft tissue construct can thereby be prefabricated to provide a variety of options for regeneration of skin and mucosa. Oral keratinocytes are a viable alternative to epidermal (skin) keratinocytes because they have unique characteristics:
- 1
Heightened growth potential in vitro, thereby necessitating a small donor site
- 2
Ability of cells to expand for coverage of larger wounds more rapidly
- 3
Secretion of pro-angiogenic factors, such as VEGF and IL-8, which enhance cell integration at graft sites
- 4
Secretion of potent antimicrobial peptides (e.g., β-defensins), which may be able to play a role in combating infections
Autogenous oral keratinocytes are harvested through the use of a small tissue punch biopsy and subsequently developed and grown on top of acellular, non-immunogenic, human cadaveric dermis (AlloDerm, LifeCell, Branchburg, NJ), thereby generating an ex vivo–produced oral mucosa equivalent (EVPOME) ( Fig. 9-3 ). AlloDerm, used as the dermal equivalent for EVPOME, has excellent handling characteristics, provides the grafts with a supple nature, and is an off-the-shelf tissue product well established in the field of oral and maxillofacial surgery. EVPOME is used as a full-thickness mucosa or skin substitute ( Fig. 9-4 ) and has keratinization characteristics identical to those of native oral tissues.
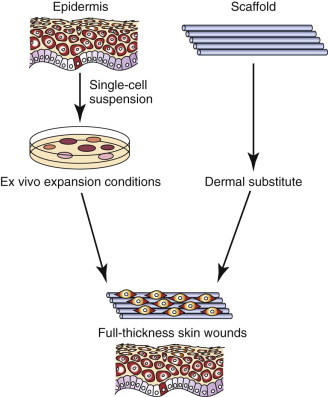
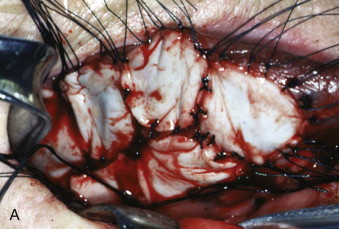
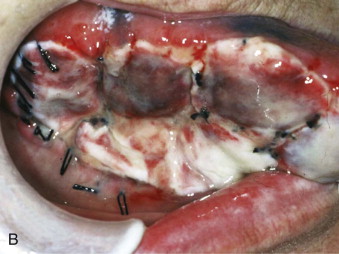
Procedure
A small, 4- to 6-mm tissue punch biopsy specimen is harvested from the oral mucosa 3 to 4 weeks (depending on the size of the grafts needed) before the desired reconstructive surgery. Palatal tissue provides a keratinized mucosa equivalent, whereas the retromolar pad or buccal mucosa provides non-keratinized mucosa equivalent. The harvested tissue is transferred into a culture medium and transported to a cGMP (current Good Manufacturing Practice) facility. The harvested sites can be closed primarily (buccal, retromolar) or have a protective collagen plug sutured into place (palate).
The manufacturing and culturing procedures for EVPOME have been described in detail elsewhere. Briefly, oral keratinocytes are dissociated by soaking the harvested biopsy tissue in 0.04% trypsin solution. The cells are grown in a serum-free culture medium. The lack of xenogeneic cells avoids any cross-contamination of the autogenous oral keratinocytes. Following growth of a sufficient number of keratinocytes over a period of 1 to 2 weeks, cells are seeded onto pieces of type IV collagen–presoaked AlloDerm (cadaveric human dermis) on the roughened side containing an intact basement membrane. For the first 4 days ( Fig. 9-5 ) a composite of keratinocytes and AlloDerm is incubated in a submerged culture. For the next 7 days the composite is raised at an air-liquid interface culture with a higher calcium concentration to promote keratinocyte differentiation ( Fig. 9-6 ). On reaching day 11 after seeding the dermis with keratinocytes, the EVPOME grafts are ready for transplantation ( Fig. 9-7 ).
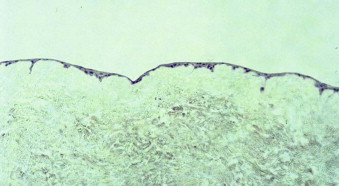
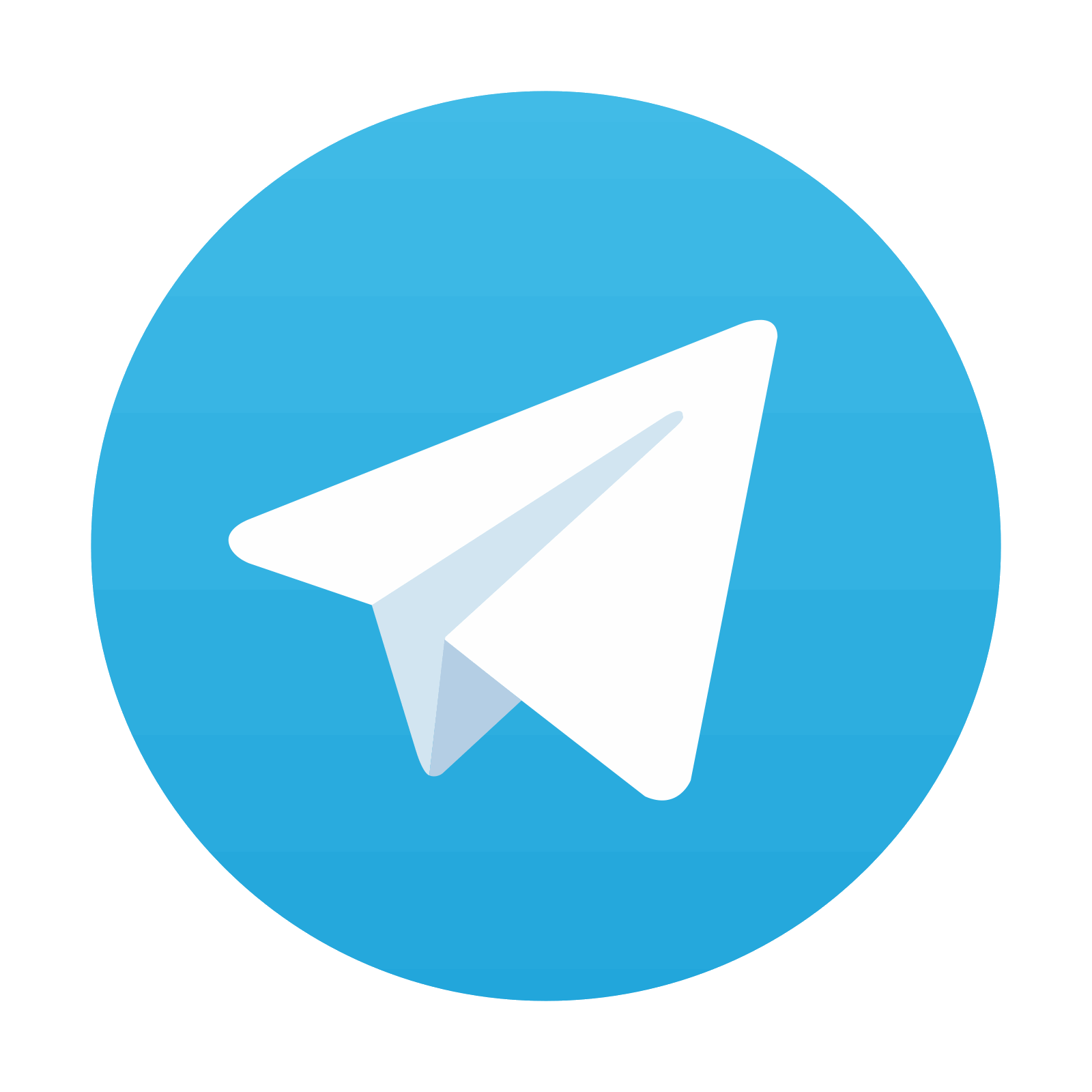
Stay updated, free dental videos. Join our Telegram channel

VIDEdental - Online dental courses
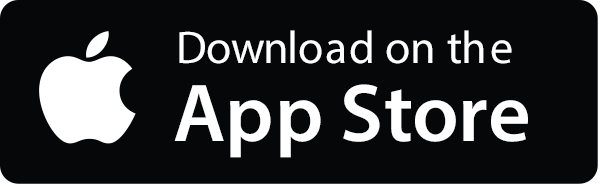
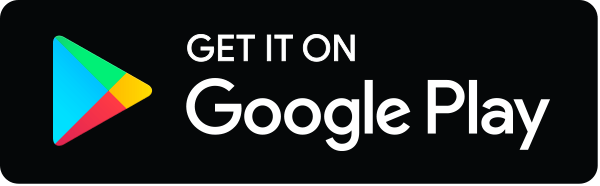