Abstract
Objective
The focus of this review is to summarize recent advances on regenerative technologies (scaffolding matrices, cell/gene therapy and biologic drug delivery) to promote reconstruction of tooth and dental implant-associated bone defects.
Methods
An overview of scaffolds developed for application in bone regeneration is presented with an emphasis on identifying the primary criteria required for optimized scaffold design for the purpose of regenerating physiologically functional osseous tissues. Growth factors and other biologics with clinical potential for osteogenesis are examined, with a comprehensive assessment of pre-clinical and clinical studies. Potential novel improvements to current matrix-based delivery platforms for increased control of growth factor spatiotemporal release kinetics are highlighting including recent advancements in stem cell and gene therapy.
Results
An analysis of existing scaffold materials, their strategic design for tissue regeneration, and use of growth factors for improved bone formation in oral regenerative therapies results in the identification of current limitations and required improvements to continue moving the field of bone tissue engineering forward into the clinical arena.
Significance
Development of optimized scaffolding matrices for the predictable regeneration of structurally and physiologically functional osseous tissues is still an elusive goal. The introduction of growth factor biologics and cells has the potential to improve the biomimetic properties and regenerative potential of scaffold-based delivery platforms for next-generation patient-specific treatments with greater clinical outcome predictability.
1
Introduction
The alveolar processes of the mandible and maxilla line the alveolus and provide structural support and maintenance for teeth as part of the periodontium, consisting of the periodontal ligament (PDL), cementum, connective tissue, and gingiva. Alveolar bone is especially susceptible to inflammation-induced bone resorption due to high rates of progressive periodontitis – a leading chronic oral inflammatory disease estimated to affect 47.2% of adults in the United States, with a prevalence of 70% for adults aged 65 years and older . Advanced periodontal disease alters alveolar bone morphology and destroys surrounding tooth-supporting tissues, thereby necessitating tooth extraction. Since the existence of alveolar bone is mutually connected to the dentition and other periodontal tissues, the alveolar ridge continues to resorb following tooth removal even if a dental implant is placed into a fresh extraction socket. Physiologically, this is caused by continuous bone remodeling in response to mechanical loading changes that occur with alterations in the applied force and strain distribution to the osseous tissue during mastication, as stipulated by Wolff’s Law . Ridge or socket preservation and augmentation using bone grafting materials is a clinically viable approach to maintain any remaining bone following tooth extraction and further condition it in preparation for dental implant placement. Sufficient bone volume, height, and width are necessary to ensure implant stability and osseointegration that can sustain optimal bone-implant contact biomechanical loading. Other dental procedures that involve grafting include maxillary sinus floor augmentation, which is employed for patients with bone loss in the posterior maxilla that houses premolar and molar teeth . Bone defects in the oral cavity resulting from trauma, chronic infection, congenital defects, or surgical resection require clinical intervention, most frequently using autologous bone grafting techniques. However, critical limitations of this approach include donor site morbidity and inadequate supply of graft tissue. Tissue engineering approaches using scaffolds alone or in combination with growth factor, cell and/or gene delivery have the potential to address existing challenges in managing bone loss and increase clinical options for controllable regeneration of intraoral osseous tissues.
2
Scaffolds
2.1
Intraoral bone grafts
An autologous bone graft is considered the gold standard due to low risk of immunogenicity or disease transmission that could be associated with an allograft (genetically different donor from the same species) or xenograft (donor from another species). Most importantly, bone transplanted from the patient is native to its host environment and readily associates with the remnant tissue, providing a pre-established population of viable cells and growth factors necessary for osteogenesis. Local sites such as the maxillary tuberosity or mandibular symphysis can be used for harvesting of small autologous grafts . Nevertheless, there are several key reasons for a critical need of alternative grafts capable of substituting the autograft: limited availability of autologous tissue for larger bone defects, donor site morbidity and potential wound-based infections, as well as prolonged operative times . Although lacking in osteogenicity, allografts and xenografts can be prepared to have osteoconductive and osteoinductive properties. Bone allografts are available as fresh/fresh-frozen, freeze-dried, or demineralized and freeze-dried. The mechanical properties of allografts derived from a living donor or cadaveric tissue are changed substantially during extensive tissue processing involving decellularization, sterilization, and preservation for clinical use . Such tissue treatment removes viable cells that are osteogenic and osteoinductive in nature, leaving behind a structurally supportive framework primarily composed of minerals and proteins – termed the extracellular matrix (ECM). The allograft ECM serves as a scaffold for osteoblasts originating from the bone defect into which the graft is placed to facilitate new bone formation. Depending on the method of processing, an allograft can also be osteoinductive if it retains the biological properties necessary to recruit mesenchymal stem cells to the site and stimulate their differentiation into osteoprogenitor cells. One example is demineralized bone matrix (DMB), which has reduced levels of calcium and phosphorus and is primarily type I collagen, but can be considered osteoinductive if it retains factors such as bone morphogenetic proteins (BMPs) and transforming growth factor-β (TGF-β) . As expected, DMB shows an increased rate of resorption relative to a mineralized bone graft during tissue remodeling in vivo. In addition, derivation of DBM involves grinding of bone to obtain particulates as opposed to processing the allograft in its native structural form, making it useful for small to moderate defects .
Xenografts offer another alternative for bone replacement in dental regeneration, with most products derived from coral, porcine, or bovine sources. A recent study comparing implant placement into sinus floors augmented with an autologous mandibular bone graft versus a commercially-available bovine xenograft found equivalent implant survival rates over an observational period of 5 years . However, implant survivability depends on many factors, including patient demographics and surgical technique, thereby warranting longer-term evaluations and more comprehensive consideration of factors that may influence the clinical outcome. Extensive meta-analysis of histomorphometric and bone graft healing time results for sinus floor augmentation described in the literature over a period of 16 years concluded that autologous bone grafts result in higher total bone volume levels compared to other bone grafting materials . Another comprehensive systematic review of treatment modalities used to evaluate dental implant survival rates in maxillary sinus grafts employed statistically robust methodology to correct for study effects. It concluded that application of grafting membranes for guided bone regeneration supplementary to a bone graft was more important for implant survival rate over factors such as which bone substitute material was selected for the surgery . These results indicate the difficulty of identifying specific factors that influence final clinical outcomes and underline the fact that there is no unified consensus on whether non-patient derived grafts can perform at the same level as autografts for not only bone regeneration but also implant performance at augmented bone sites. Each case is patient-specific and requires thorough consideration of all contributing factors, including the health of the patient’s native bone and its suitability for grafting procedures.
2.2
Natural and synthetic matrices
In addition to standard grafting procedures using bone-derived materials, a number of natural and synthetic materials have become commercially available for use in oral surgery. Scaffolds that are architecturally and/or biologically compatible for bone regeneration are frequently based on one or more of the bone’s naturally-occurring proteins or minerals, including organic (predominantly collagen type I) and inorganic (hydroxyapatite, a calcium phosphate mineral) components . Calcium phosphate (CaP) materials are subdivided into ceramics and cements, which vary in their rate of in vivo degradation, structure, and mechanical strength. Common synthetic CaP bone substitutes include hydroxyapatite (HA) ceramics, β-tricalcium phosphate (β-TCP) cements, and biphasic calcium phosphates (BCPs) . Fragility and poor fatigue resistance of these ceramics and cements requires their use at non-load bearing bone replacement sites or as coatings on load-bearing metal implants for increased bone-to-implant contact. Coating a dental implant surface (i.e., titanium, stainless steel, or an cobalt-chrome alloy) with CaP-derivatives has been extensively investigated using various surface coating deposition techniques to improve implant stability and rate of osseointegration . In addition to containing minerals native to osseous tissue, these biomaterials retain an interconnected porous architecture – allowing adequate space through increased surface area for bone ingrowth via cell infiltration, blood vessel formation, nutrient/oxygen transport, and waste elimination. Ongoing studies are being performed to determine the optimum porosity for bone ingrowth and corresponding bone substitute resorption rate, since an ideal regenerative scenario would consist of a biomaterial resorption rate timed with new osseous tissue ingrowth . Likewise, there are ongoing investigations to confirm the utility of using CaP bone substitutes for implant coating. For example, recent studies of hydroxyapatite coatings on titanium cups for orthopedic-based implants such as femoral stems did not show significant differences between HA-coated and non-coated stems and may not constitute a clinical advantage .
Bioactive glass (BG), a silicon oxide with substituted calcium first developed in the 1960s by Professor Larry Hench, is a biocompatible glass-ceramic material approved by the US Food and Drug Administration (FDA) for use as a synthetic intraoral bone graft (termed 45S5 Bioglass ® ). Upon exposure to aqueous solutions the highly reactive surface converts to a gel layer that mineralizes to form a osteoconductive hydroxycarbonate apatite layer that chemically binds with osseous tissues . Bioglass ® also has a reported elastic modulus of 35 GPa that is similar to that of cortical bone ( E longitudinal = ∼14–20 GPa) . Comparatively, the elastic moduli of HA single crystals and β-TCP are in the range of 54–79 GPa and 120–162 GPa, respectively . This makes BG an attractive option for metal implant coating, reducing the potential for stress shielding and subsequent bone resorption which occurs with decreased bone loading . To date, studies using dental implants coated with BGs have not conclusively shown significant increases in osseointegration relative to other coatings such as HA . However, novel exploratory combinations for dental implant coatings using BG are promising: one example is the incorporation of BGs with HA and the polymer poly(lactide- co -glycolide) (PLGA), which indicates rapid bone-like apatite formation in vitro, with potential antimicrobial activity on oral bacteria .
Polymeric materials that have been commercialized or are currently under investigation for intraoral bone regeneration are either naturally- or synthetically-derived. Natural materials that consist of polymeric networks (i.e., collagen, alginate) have been extensively investigated as composite materials with other bone replacement grafts, including β-TCP and HA . Major advantages of a tissue-sourced polymer such as collagen include its biocompatibility, biodegradability, and ability to readily bind growth factors critical for osteoinduction, including BMPs. Currently, collagen is the most commercially-available natural polymer on the market for use in periodontal bone regeneration as a sponge, membrane, or in particulate form combined with other bone grafts . It is expected that future research will continue to focus on the development of a more diverse array of naturally-derived, fully-resorbable polymeric bone grafts combined with non-immunogenic materials such as alginate and chitosan that can be engineered for growth factor, cell, and/or gene delivery. Such delivery devices may also include synthetically-derived resorbable materials whose physical, mechanical, and degradation properties can be more easily controlled via polymer chemical composition and molecular weight. Historically, oral surgery has utilized non-resorbable synthetic membranes as cell-occlusive barriers for guided bone regeneration, among which the most broadly used material has been expanded polytetrafluoroethylene (ePTFE) . Unnecessary patient discomfort and added cost of a follow-up visit to remove the membrane has increased demand for resorbable alternatives. Examples of widely-investigated resorbable polymers for applications in bone regeneration include poly-α-hydroxy esters such as polyglycolic acid (PGA), polylactic acid (PLA), and PLGA. The ease of processing synthetic materials enables their fabrication into a variety of structurally-diverse forms, including thin films, meshes, fibers, and porous foams. An existing disadvantage of these materials is their bulk erosion in vivo due to hydrolysis that can induce foreign body reactions to acidic polymer degradation products, increasing the potential for fibrous tissue encapsulation during wound healing. Although studies of PLGA utility for guided bone regeneration are still limited, there is evidence that its use as a barrier membrane results in alveolar bone regeneration that is on par with that observed using a collagen-based membrane . Other copolymer combinations that yield useful properties for osseous tissue regeneration include PLGA with poly(ethylene glycol) (PEG), a widely used biocompatible hydrophilic drug delivery carrier. PEG-PLGA thermo-sensitive copolymers can be encapsulated with osteoinductive factors and polymerized into a gel-like structure for delivery into an osseous defect, possibly in combination with other mechanically durable bone grafts . PCL is another biodegradable polymer with significant research indicating its suitability for bone regeneration. An inert material, it shows increased osteoblast adhesion, spreading, and proliferation when coated with CaP or HA , and multiple studies have indicated PCL’s potential to promote alveolar bone formation in periodontal defects . With greater emphasis placed on materials that can be used for exogenous factor delivery to accelerate and improve existing periodontal tissue defect treatments, polymers such as PCL and PEG which are already FDA-approved as drug delivery devices are expected to be more extensively investigated in pre-clinical models of intraoral bone regeneration in the coming years.
2.3
Engineering scaffolds for intraoral bone regeneration
The development of scaffolds that are optimal for regeneration of osseous tissues requires a design strategy which adheres to established knowledge of the mechanical, chemical, structural, and biological properties of natural bone that make it a functional entity. Therefore, key considerations in such scaffold design include: (1) biocompatibility/non-toxic degradation; (2) bioactivity, enabling cell interaction with material surface; (3) maintenance of a 3-D shape after implantation; (4) adequate porosity and pore diameter/distribution/orientation; (5) mechanical properties similar to tissue targeted for regeneration (i.e., Young’s modulus); (6) degradation mechanics (i.e., bulk erosion, surface erosion); (7) degradation rate, ideally matching the rate of tissue regeneration; and (8) osteoconductive/inductive and angiogenic factors to influence infiltrating cell populations and promote blood vessel invasion .
While a broad range of instructive carrier materials in various forms have been investigated for bone regeneration, the addition of cells and application of growth factor delivery strategies can significantly influence the regenerative outcome by engineering the environment that closely matches that of the target tissue in its native state (see Fig. 1 ). Current strategies include the use of bone-marrow stromal cells and stem cell varieties including mesenchymal (MSCs), adipose-derived (ADSCs) and induced pluripotent (iPSCs). Analyses of cell-specific markers and transcription factors such as Runx2, alkaline phosphatase (ALP), osteocalcin, osteopontin, and osteonectin allow for the determination of osteogenicity during stem cell differentiation into bone-derived cells . Clinically-applicable cell therapy is focused on the use of patient-derived stem cells that are undifferentiated, given that terminally-differentiated cells are difficult to expand ex vivo relative to more highly proliferative stem/progenitor cells. Likewise, the use of stem cells allows for a more completely physiological repair process that involves the differentiation of MSCs or iPSCs not only into bone-derived cells but also cell types involved in neovascularization, such as endothelial cells. However, despite significant progress and tremendous potential in cell therapy, critical challenges remain in transitioning the use of iPSCs into clinically-applicable approaches. Since the creation of iPSCs involves the reprogramming of somatic cells via transcription factors to produce cells with embryonic stem cell-like properties, there is risk of epigenetic and genetic defect accumulations, immunogenic responses, or tumor formations. While the immunogenic response can be mediated through use of a patient’s native somatic cells, overexpression of transcription factors or presence of partially-reprogrammed iPSCs are known to cause teratoma formation, requiring significant efforts to address these safety concerns prior to the consideration of iPSCs for patient-based treatments .

With advancements in cell therapy, there has been a simultaneous increase in novel scaffold fabrication techniques that emphasize greater control over surface topography, internal microstructure, and pore interconnectivity. Traditionally, porous scaffolds have been widely explored as bone graft substitutes for cell attachment given the importance of allowing adequate room for tissue ingrowth and vascularization (i.e., pore size of 150–500 μm) . While natural materials retain their bioactivity, synthetic non-immunogenic materials have several advantages for development of clinically-translatable scaffolds, including added flexibility in manufacturing, reproducibility, sterilization, and storage times. Electrospinning and solid freeform fabrication (SFF) are two scaffold fabrication techniques which allow increased control over scaffold morphology: Electrospinning is a polymer-processing technique used for creating polymer fibers on the nano and micron scale to influence cell behavior through structural and physical cues that mimic ECM architecture. In addition to allowing control over a variety of parameters that determine fiber dimension, density, and porosity, electrospinning can readily be used for mass production of fiber-based scaffolds . More recently, 3-D printing technology has been adapted for use in bone tissue engineering via solid freeform fabrication (SFF), a rapid prototyping technique. This process consists of developing a computer-aided design (CAD) file that specifies the exact dimensional features of the desired scaffold which is then transferred to a 3-D printer that reproduces the file to yield a printed version of the design with structural integrity. Selective laser sintering (SLS) is an example of a process that creates objects layer-by-layer using polymeric, ceramic, or metal powders that the machine sinters. During sintering, the powder is heated below the melting point, causing its particle boundaries to fuse together at locations dictated by the CAD-based file. When creating a porous material, the sizes and characteristics of the individual pores within the material are limited by the machine’s resolution and ability to support a specific printing material . Studies using SLS for bone regeneration have focused on PCL-based printed scaffolds, showing that these are mechanically-appropriate to support bone tissue formation and can be used as BMP-7 and BMP-2 growth factor carriers following biofunctionalization . This technology is especially applicable for clinically-based studies given that patient-specific anatomical bone defects can be obtained using computed tomography (CT) scans and reproduced to yield a scaffold with appropriate structural dimensions. A recent publication by Park et al. proposes a potential future application of image-based PCL SFF-based scaffolds for clinical periodontal regeneration (see Fig. 2 ), indicating that further pre-clinical investigation and verification of these technologies will bring them closer to translation into clinical practice .

While novel methods of scaffold development and cell therapy are being explored for bone regeneration, there is already an established array of clinically-applicable therapeutic factors and delivery strategies. In order to better understand existing clinical applications and the nature of ongoing pre-clinical studies, the remainder of this review focuses on the areas of growth factor delivery for oral bone regeneration and highlights some of the key studies that have moved previously experimental therapies into today’s mainstream clinical applications in periodontal regeneration.
2
Scaffolds
2.1
Intraoral bone grafts
An autologous bone graft is considered the gold standard due to low risk of immunogenicity or disease transmission that could be associated with an allograft (genetically different donor from the same species) or xenograft (donor from another species). Most importantly, bone transplanted from the patient is native to its host environment and readily associates with the remnant tissue, providing a pre-established population of viable cells and growth factors necessary for osteogenesis. Local sites such as the maxillary tuberosity or mandibular symphysis can be used for harvesting of small autologous grafts . Nevertheless, there are several key reasons for a critical need of alternative grafts capable of substituting the autograft: limited availability of autologous tissue for larger bone defects, donor site morbidity and potential wound-based infections, as well as prolonged operative times . Although lacking in osteogenicity, allografts and xenografts can be prepared to have osteoconductive and osteoinductive properties. Bone allografts are available as fresh/fresh-frozen, freeze-dried, or demineralized and freeze-dried. The mechanical properties of allografts derived from a living donor or cadaveric tissue are changed substantially during extensive tissue processing involving decellularization, sterilization, and preservation for clinical use . Such tissue treatment removes viable cells that are osteogenic and osteoinductive in nature, leaving behind a structurally supportive framework primarily composed of minerals and proteins – termed the extracellular matrix (ECM). The allograft ECM serves as a scaffold for osteoblasts originating from the bone defect into which the graft is placed to facilitate new bone formation. Depending on the method of processing, an allograft can also be osteoinductive if it retains the biological properties necessary to recruit mesenchymal stem cells to the site and stimulate their differentiation into osteoprogenitor cells. One example is demineralized bone matrix (DMB), which has reduced levels of calcium and phosphorus and is primarily type I collagen, but can be considered osteoinductive if it retains factors such as bone morphogenetic proteins (BMPs) and transforming growth factor-β (TGF-β) . As expected, DMB shows an increased rate of resorption relative to a mineralized bone graft during tissue remodeling in vivo. In addition, derivation of DBM involves grinding of bone to obtain particulates as opposed to processing the allograft in its native structural form, making it useful for small to moderate defects .
Xenografts offer another alternative for bone replacement in dental regeneration, with most products derived from coral, porcine, or bovine sources. A recent study comparing implant placement into sinus floors augmented with an autologous mandibular bone graft versus a commercially-available bovine xenograft found equivalent implant survival rates over an observational period of 5 years . However, implant survivability depends on many factors, including patient demographics and surgical technique, thereby warranting longer-term evaluations and more comprehensive consideration of factors that may influence the clinical outcome. Extensive meta-analysis of histomorphometric and bone graft healing time results for sinus floor augmentation described in the literature over a period of 16 years concluded that autologous bone grafts result in higher total bone volume levels compared to other bone grafting materials . Another comprehensive systematic review of treatment modalities used to evaluate dental implant survival rates in maxillary sinus grafts employed statistically robust methodology to correct for study effects. It concluded that application of grafting membranes for guided bone regeneration supplementary to a bone graft was more important for implant survival rate over factors such as which bone substitute material was selected for the surgery . These results indicate the difficulty of identifying specific factors that influence final clinical outcomes and underline the fact that there is no unified consensus on whether non-patient derived grafts can perform at the same level as autografts for not only bone regeneration but also implant performance at augmented bone sites. Each case is patient-specific and requires thorough consideration of all contributing factors, including the health of the patient’s native bone and its suitability for grafting procedures.
2.2
Natural and synthetic matrices
In addition to standard grafting procedures using bone-derived materials, a number of natural and synthetic materials have become commercially available for use in oral surgery. Scaffolds that are architecturally and/or biologically compatible for bone regeneration are frequently based on one or more of the bone’s naturally-occurring proteins or minerals, including organic (predominantly collagen type I) and inorganic (hydroxyapatite, a calcium phosphate mineral) components . Calcium phosphate (CaP) materials are subdivided into ceramics and cements, which vary in their rate of in vivo degradation, structure, and mechanical strength. Common synthetic CaP bone substitutes include hydroxyapatite (HA) ceramics, β-tricalcium phosphate (β-TCP) cements, and biphasic calcium phosphates (BCPs) . Fragility and poor fatigue resistance of these ceramics and cements requires their use at non-load bearing bone replacement sites or as coatings on load-bearing metal implants for increased bone-to-implant contact. Coating a dental implant surface (i.e., titanium, stainless steel, or an cobalt-chrome alloy) with CaP-derivatives has been extensively investigated using various surface coating deposition techniques to improve implant stability and rate of osseointegration . In addition to containing minerals native to osseous tissue, these biomaterials retain an interconnected porous architecture – allowing adequate space through increased surface area for bone ingrowth via cell infiltration, blood vessel formation, nutrient/oxygen transport, and waste elimination. Ongoing studies are being performed to determine the optimum porosity for bone ingrowth and corresponding bone substitute resorption rate, since an ideal regenerative scenario would consist of a biomaterial resorption rate timed with new osseous tissue ingrowth . Likewise, there are ongoing investigations to confirm the utility of using CaP bone substitutes for implant coating. For example, recent studies of hydroxyapatite coatings on titanium cups for orthopedic-based implants such as femoral stems did not show significant differences between HA-coated and non-coated stems and may not constitute a clinical advantage .
Bioactive glass (BG), a silicon oxide with substituted calcium first developed in the 1960s by Professor Larry Hench, is a biocompatible glass-ceramic material approved by the US Food and Drug Administration (FDA) for use as a synthetic intraoral bone graft (termed 45S5 Bioglass ® ). Upon exposure to aqueous solutions the highly reactive surface converts to a gel layer that mineralizes to form a osteoconductive hydroxycarbonate apatite layer that chemically binds with osseous tissues . Bioglass ® also has a reported elastic modulus of 35 GPa that is similar to that of cortical bone ( E longitudinal = ∼14–20 GPa) . Comparatively, the elastic moduli of HA single crystals and β-TCP are in the range of 54–79 GPa and 120–162 GPa, respectively . This makes BG an attractive option for metal implant coating, reducing the potential for stress shielding and subsequent bone resorption which occurs with decreased bone loading . To date, studies using dental implants coated with BGs have not conclusively shown significant increases in osseointegration relative to other coatings such as HA . However, novel exploratory combinations for dental implant coatings using BG are promising: one example is the incorporation of BGs with HA and the polymer poly(lactide- co -glycolide) (PLGA), which indicates rapid bone-like apatite formation in vitro, with potential antimicrobial activity on oral bacteria .
Polymeric materials that have been commercialized or are currently under investigation for intraoral bone regeneration are either naturally- or synthetically-derived. Natural materials that consist of polymeric networks (i.e., collagen, alginate) have been extensively investigated as composite materials with other bone replacement grafts, including β-TCP and HA . Major advantages of a tissue-sourced polymer such as collagen include its biocompatibility, biodegradability, and ability to readily bind growth factors critical for osteoinduction, including BMPs. Currently, collagen is the most commercially-available natural polymer on the market for use in periodontal bone regeneration as a sponge, membrane, or in particulate form combined with other bone grafts . It is expected that future research will continue to focus on the development of a more diverse array of naturally-derived, fully-resorbable polymeric bone grafts combined with non-immunogenic materials such as alginate and chitosan that can be engineered for growth factor, cell, and/or gene delivery. Such delivery devices may also include synthetically-derived resorbable materials whose physical, mechanical, and degradation properties can be more easily controlled via polymer chemical composition and molecular weight. Historically, oral surgery has utilized non-resorbable synthetic membranes as cell-occlusive barriers for guided bone regeneration, among which the most broadly used material has been expanded polytetrafluoroethylene (ePTFE) . Unnecessary patient discomfort and added cost of a follow-up visit to remove the membrane has increased demand for resorbable alternatives. Examples of widely-investigated resorbable polymers for applications in bone regeneration include poly-α-hydroxy esters such as polyglycolic acid (PGA), polylactic acid (PLA), and PLGA. The ease of processing synthetic materials enables their fabrication into a variety of structurally-diverse forms, including thin films, meshes, fibers, and porous foams. An existing disadvantage of these materials is their bulk erosion in vivo due to hydrolysis that can induce foreign body reactions to acidic polymer degradation products, increasing the potential for fibrous tissue encapsulation during wound healing. Although studies of PLGA utility for guided bone regeneration are still limited, there is evidence that its use as a barrier membrane results in alveolar bone regeneration that is on par with that observed using a collagen-based membrane . Other copolymer combinations that yield useful properties for osseous tissue regeneration include PLGA with poly(ethylene glycol) (PEG), a widely used biocompatible hydrophilic drug delivery carrier. PEG-PLGA thermo-sensitive copolymers can be encapsulated with osteoinductive factors and polymerized into a gel-like structure for delivery into an osseous defect, possibly in combination with other mechanically durable bone grafts . PCL is another biodegradable polymer with significant research indicating its suitability for bone regeneration. An inert material, it shows increased osteoblast adhesion, spreading, and proliferation when coated with CaP or HA , and multiple studies have indicated PCL’s potential to promote alveolar bone formation in periodontal defects . With greater emphasis placed on materials that can be used for exogenous factor delivery to accelerate and improve existing periodontal tissue defect treatments, polymers such as PCL and PEG which are already FDA-approved as drug delivery devices are expected to be more extensively investigated in pre-clinical models of intraoral bone regeneration in the coming years.
2.3
Engineering scaffolds for intraoral bone regeneration
The development of scaffolds that are optimal for regeneration of osseous tissues requires a design strategy which adheres to established knowledge of the mechanical, chemical, structural, and biological properties of natural bone that make it a functional entity. Therefore, key considerations in such scaffold design include: (1) biocompatibility/non-toxic degradation; (2) bioactivity, enabling cell interaction with material surface; (3) maintenance of a 3-D shape after implantation; (4) adequate porosity and pore diameter/distribution/orientation; (5) mechanical properties similar to tissue targeted for regeneration (i.e., Young’s modulus); (6) degradation mechanics (i.e., bulk erosion, surface erosion); (7) degradation rate, ideally matching the rate of tissue regeneration; and (8) osteoconductive/inductive and angiogenic factors to influence infiltrating cell populations and promote blood vessel invasion .
While a broad range of instructive carrier materials in various forms have been investigated for bone regeneration, the addition of cells and application of growth factor delivery strategies can significantly influence the regenerative outcome by engineering the environment that closely matches that of the target tissue in its native state (see Fig. 1 ). Current strategies include the use of bone-marrow stromal cells and stem cell varieties including mesenchymal (MSCs), adipose-derived (ADSCs) and induced pluripotent (iPSCs). Analyses of cell-specific markers and transcription factors such as Runx2, alkaline phosphatase (ALP), osteocalcin, osteopontin, and osteonectin allow for the determination of osteogenicity during stem cell differentiation into bone-derived cells . Clinically-applicable cell therapy is focused on the use of patient-derived stem cells that are undifferentiated, given that terminally-differentiated cells are difficult to expand ex vivo relative to more highly proliferative stem/progenitor cells. Likewise, the use of stem cells allows for a more completely physiological repair process that involves the differentiation of MSCs or iPSCs not only into bone-derived cells but also cell types involved in neovascularization, such as endothelial cells. However, despite significant progress and tremendous potential in cell therapy, critical challenges remain in transitioning the use of iPSCs into clinically-applicable approaches. Since the creation of iPSCs involves the reprogramming of somatic cells via transcription factors to produce cells with embryonic stem cell-like properties, there is risk of epigenetic and genetic defect accumulations, immunogenic responses, or tumor formations. While the immunogenic response can be mediated through use of a patient’s native somatic cells, overexpression of transcription factors or presence of partially-reprogrammed iPSCs are known to cause teratoma formation, requiring significant efforts to address these safety concerns prior to the consideration of iPSCs for patient-based treatments .
With advancements in cell therapy, there has been a simultaneous increase in novel scaffold fabrication techniques that emphasize greater control over surface topography, internal microstructure, and pore interconnectivity. Traditionally, porous scaffolds have been widely explored as bone graft substitutes for cell attachment given the importance of allowing adequate room for tissue ingrowth and vascularization (i.e., pore size of 150–500 μm) . While natural materials retain their bioactivity, synthetic non-immunogenic materials have several advantages for development of clinically-translatable scaffolds, including added flexibility in manufacturing, reproducibility, sterilization, and storage times. Electrospinning and solid freeform fabrication (SFF) are two scaffold fabrication techniques which allow increased control over scaffold morphology: Electrospinning is a polymer-processing technique used for creating polymer fibers on the nano and micron scale to influence cell behavior through structural and physical cues that mimic ECM architecture. In addition to allowing control over a variety of parameters that determine fiber dimension, density, and porosity, electrospinning can readily be used for mass production of fiber-based scaffolds . More recently, 3-D printing technology has been adapted for use in bone tissue engineering via solid freeform fabrication (SFF), a rapid prototyping technique. This process consists of developing a computer-aided design (CAD) file that specifies the exact dimensional features of the desired scaffold which is then transferred to a 3-D printer that reproduces the file to yield a printed version of the design with structural integrity. Selective laser sintering (SLS) is an example of a process that creates objects layer-by-layer using polymeric, ceramic, or metal powders that the machine sinters. During sintering, the powder is heated below the melting point, causing its particle boundaries to fuse together at locations dictated by the CAD-based file. When creating a porous material, the sizes and characteristics of the individual pores within the material are limited by the machine’s resolution and ability to support a specific printing material . Studies using SLS for bone regeneration have focused on PCL-based printed scaffolds, showing that these are mechanically-appropriate to support bone tissue formation and can be used as BMP-7 and BMP-2 growth factor carriers following biofunctionalization . This technology is especially applicable for clinically-based studies given that patient-specific anatomical bone defects can be obtained using computed tomography (CT) scans and reproduced to yield a scaffold with appropriate structural dimensions. A recent publication by Park et al. proposes a potential future application of image-based PCL SFF-based scaffolds for clinical periodontal regeneration (see Fig. 2 ), indicating that further pre-clinical investigation and verification of these technologies will bring them closer to translation into clinical practice .
While novel methods of scaffold development and cell therapy are being explored for bone regeneration, there is already an established array of clinically-applicable therapeutic factors and delivery strategies. In order to better understand existing clinical applications and the nature of ongoing pre-clinical studies, the remainder of this review focuses on the areas of growth factor delivery for oral bone regeneration and highlights some of the key studies that have moved previously experimental therapies into today’s mainstream clinical applications in periodontal regeneration.
3
Growth factors and protein delivery
Growth factor delivery (GFD) is of critical importance in mediating the scaffold environment and subsequent cellular response. Growth factors are soluble polypeptides that bind to cell membrane receptors and influence cellular function, and the inclusion of osteoinductive/conductive factors (BMP, TGF-β, IGF, FGF-2, PDGF) guides the cell differentiation and tissue formation process in bone regenerative therapies. Likewise, vascularization is vital for the sustainability of newly-formed tissue, and necessitates the inclusion of GFs with angiogenic properties (PDGF, VEGF, FGF-2, and TGF-β). Scaffold-based GFD as opposed to bolus injection of the therapeutic factors into the defect site has several advantages, including the potential for improved control over GF release kinetics and localization. A major challenge in GFD involves the need for release profiles that mimic those present during natural tissue repair or morphogenesis. Sequential or simultaneous spatiotemporal delivery of multiple GFs can be achieved using scaffolds based on the therapeutic time window during which GF delivery is optimal for tissue regeneration, and can involve the combination of factors involved in both tissue formation and angiogenesis. For example, sequential delivery of GF combinations consisting of BMP-2 and TGF-β or VEGF and BMP showed increased bone formation when compared to single GFD . GF rate of release is governed by how it is bound to the scaffold, which can involve (1) mixing the GF with scaffold particles, (2) physical encapsulation within the scaffold, (3) chemical immobilization, and (4) affinity-based binding. Current clinical application of GFs for bone regeneration typically involves the use of biomaterial carriers, yet the factor is usually mixed in and physically adsorbed onto the graft particles and lacks more sophisticated modes of delivery that would enable spatiotemporal control over release kinetics or dual GFD. Other modes of delivery currently investigated in pre-clinical studies are promising: Physical encapsulation of GFs within the scaffold, for example, can be achieved using polymeric microparticles (1–100 μm) with varying surface areas through which the encapsulated GF diffuses at a rate that is dependent on carrier particle size, with a larger size leading to slower diffusion. GFs can also be conjugated on the scaffold surface via covalent immobilization, while heparin-binding GFs such as BMP-2 can be presented using affinity-based binding by conjugating heparin to biomaterials . These modes of GFD have potential for future applications in clinical studies, allowing for a broad range of release profiles and controlled spatiotemporal presentation of multiple GFs using carriers that serve as temporary support structures for osseous tissue engineering strategies.
Growth factors and proteins act locally on the activity of periodontal cell populations to modulate bone formation and enhance the regenerative response (see Table 1 ). A diverse range of bone matrix-based proteins have been isolated and are delivered alone or in combination with a synthetically- or naturally-derived bone graft. An overview of some of the factors and proteins that are featured most prominently in oral-based surgery or currently being investigated in pre-clinical (see Table 2 ) and clinical studies (see Table 3 ) is presented here.
Growth factor | Cell type | Effect | Studies |
---|---|---|---|
PDGF | Cementoblasts | • Increased DNA synthesis and osteopontin mRNA expression | |
Dental follicle cells | • Stimulated DNA synthesis and expression of CSF-1 and MCP-1 | ||
Gingival fibroblasts | • Increased mitosis • No increase in proliferation or chemotaxis |
||
Osteoblasts | • Increased proliferation and interleukin-6 transcription • Inhibited differentiation • Blocked osteopontin, osteonectin |
||
PDL cells | • Stimulated proliferation (with or without allograft) • Induced matrix synthesis and increased cell migration and mitosis |
||
BMP2 | Cementoblasts | • Inhibited differentiation and mineralization | |
Dental follicle cells | • Stimulated osteoblast/cementoblast differentiation • Increased mineralization and ALP |
||
Gingival fibroblasts | • Decreased mitosis • At high doses, inhibited mineralization and OCN |
||
Osteoblasts | • Increased proliferation, mineralization, and expression of ALP and OCN | ||
PDL cells | • Increased mineralization and expression of mineralization markers • At doses >10 ng/mL, induced apoptosis/cytotoxicity • Stimulated osteoblast differentiation • Decreased mitosis |
||
BMP-7 | Cementoblasts | • Increased mineralization and mineralized tissue markers | |
Dental follicle cells | • Increased mineralization and ALP expression | ||
Osteoblasts | • Increased proliferation, mineralization, and expression of ALP and OCN | ||
PDL cells | • Reduced proliferation • Induced ALP |
||
FGF-2 | Cementoblasts | • Stimulated DNA synthesis • Decreased mineralization and expression of OCN • Modulated expression of OPN |
|
Dental follicle cells | • Stimulated DNA synthesis and expression of CSF-1 and MCP-1 | ||
Gingival epithelial cells | • Increased proliferation | ||
Gingival fibroblasts | • Increased proliferation | ||
Osteoblasts | • Promoted differentiation • Induced proliferation • Decreased mineralization gene expression |
||
PDL cells | • Increased proliferation (alone and combined with DFDBA or FDBA), migration, and extracellular matrix production • Maintained differentiation potential • Stimulated OPN • Inhibited ALP expression, mineralization, and OCN |
||
GDF-5 | CT fibroblasts | • Increased proliferation | |
Dental follicle cells | • Reduced ALP activity | ||
Osteoblasts | • Increased early differentiation and matrix production • Modulated proliferation |
||
PDL cells | • Increased proliferation and matrix synthesis • Decreased ALP activity |
||
Teriparatide | PDL cells Osteoblasts |
• Modified proliferation & survival and expression of mineralized markers (dependent on maturation state) |
Growth factor | Model | Animal | Results | Studies |
---|---|---|---|---|
PDGF-BB | Furcation defect | Canine | • Stimulated PDL formation (early stage) • Promoted periodontal regeneration (late stage) |
|
GBR at implants | Canine | • With IGF-1, significantly increased histologic bone-implant contact and peri-implant bone fill • With IGF-1, increased early (3 week) bone formation at immediate implants • With ePTFE membrane and IGF-1, increased bone gain and histologic parameters versus membrane alone or membrane + DFDBA |
||
Periodontal defect | Canine | • After flap surgery, increased new bone, cementum and PDL • With IGF-1, promotes periodontal regeneration |
||
Non-human primate | • Significantly increased new attachment | |||
Ridge augmentation | Canine | • With block graft, increased histologic bone formation • With bone mineral and collagen membrane, supports lateral bone formation • With biphasic calcium phosphate and collagen membrane, supports GBR • With xenograft scaffold, promoted bone regeneration similar in quality to native bone • With xenograft, improved radiographic results when used without collagen membrane |
||
rhBMP-2 | Extraction socket | Rat | • Increased speed and quantity of bone formation • Induced proliferation and differentiation of mesenchymal cells |
|
Furcation defect | Feline | • Early ankylosis may resolve with polymer carrier | ||
GBR at implants | Canine | • Resorbable and ePTFE membranes delay early (1 month) bone formation but may result in increased or similar 3-month bone formation vs. no membrane • Increased bone augmentation for implants placed in extraction sockets and for implant-site circumferential and fenestration defects • BMP2-coated implants provide increased bone formation, histologic bone apposition, and osseointegration • Improved late (3-month) bone formation for ACS versus PLGA carrier |
||
Periodontal defect | Non-human primate | • Increased bone and cementum regeneration | ||
Canine | • Increased quantity and speed of bone formation • Bone quantity formed correlated with residual bone height • Limited cementum regeneration • Induced ankylosis and root resorption • No benefit for calcium phosphate cement carrier |
|||
Ridge augmentation | Rat | • Significant horizontal and vertical bone augmentation • ePTFE membranes improve bone contour • Increased bone formation with ACS+ bone graft material • Potential carriers: absorbable collagen sponge, hyaluronic acid polymer, • collagen-calcium hydroxyapatite-TCP complex, PLGA/gelatin sponge |
||
Canine | • Increased histologic and radiographic bone formation, + – bone graft • Increased incidence of seromas and wound failure • Possible decreased bone quality when combined with bone graft • With xenograft block, supported bone formation • Late-stage implant stability comparable to native bone |
|||
Non-human primate | • Increased ridge width and bone quality in TCP/HA/ACS and CaP cement carriers |
|||
Sinus augmentation | Canine | • Enhanced histologic bone formation | ||
Rabbit | • Increased bone volume for collagenated BCP/BCP carriers | |||
Goat | • Increased radiographic bone formation | |||
Non-human primate | • Increased vertical bone gain | |||
Mini-pig | • BMP-2 coatings did not improve peri-implant bone gain | |||
BMP-4 | Ridge augmentation | Rat | • Improved bone quality and comparable quantity versus BMP-2 | |
BMP-7 | Furcation defect | Canine | • Significantly increased histologic bone, cementum, and new attachment in class III furcations | |
GBR at implants | Canine | • Implant-coating applications | ||
Non-human primate | • Stimulated cementum formation | |||
Ridge augmentation | Rat | • Increased bone formation in xenograft block versus control | ||
Sinus augmentation | Non-human primate | • Comparable radiographic and histologic bone formation and residual lateral wall defect reduction versus bone graft | ||
Mini-pig | • With xenograft, increases speed and quality of osseointegration at simultaneous implants | |||
Socket augmentation | Rabbit | • Histologic increased speed of healing by 4–6 weeks • Significantly increased ALP activity and calcium |
||
FGF-2 | Furcation defect | Canine | • Significantly increased regeneration of cementum, PDL, and bone vs. controls | |
GDF-5 | GBR at implants | Canine | • Improves peri-implant GBR (β-TCP) • GDF-5 coating may increase bone formation (dose-dependent) |
|
Implant coating | Rabbit | • Improved implant stability as determined by pull-out test | ||
Periodontal defect | Canine | Significantly increased perio. regeneration in PLGA (dose-dependent), β-TCP, ACS carriers, with bone formation for β-TCP stable up to 24 weeks • Beta-TCP/PLGA carrier may cause ankylosis |
||
Non-human primate | • Supported periodontal regeneration with β-TCP carrier | |||
Ridge augmentation | Canine | • With xenograft block, supported bone regeneration | ||
Sinus augmentation | Mini-pigs | • Enhanced bone formation with β-TCP | ||
Teriparatide | Extraction socket | Osteopenic rats | • Increased bone mineral density and anabolic effects | |
GBR at implants | Canine | • Significantly improved bone formation | ||
Periodontal defect | Ovarectimized rats | • Preventative effects on periodontal bone loss |
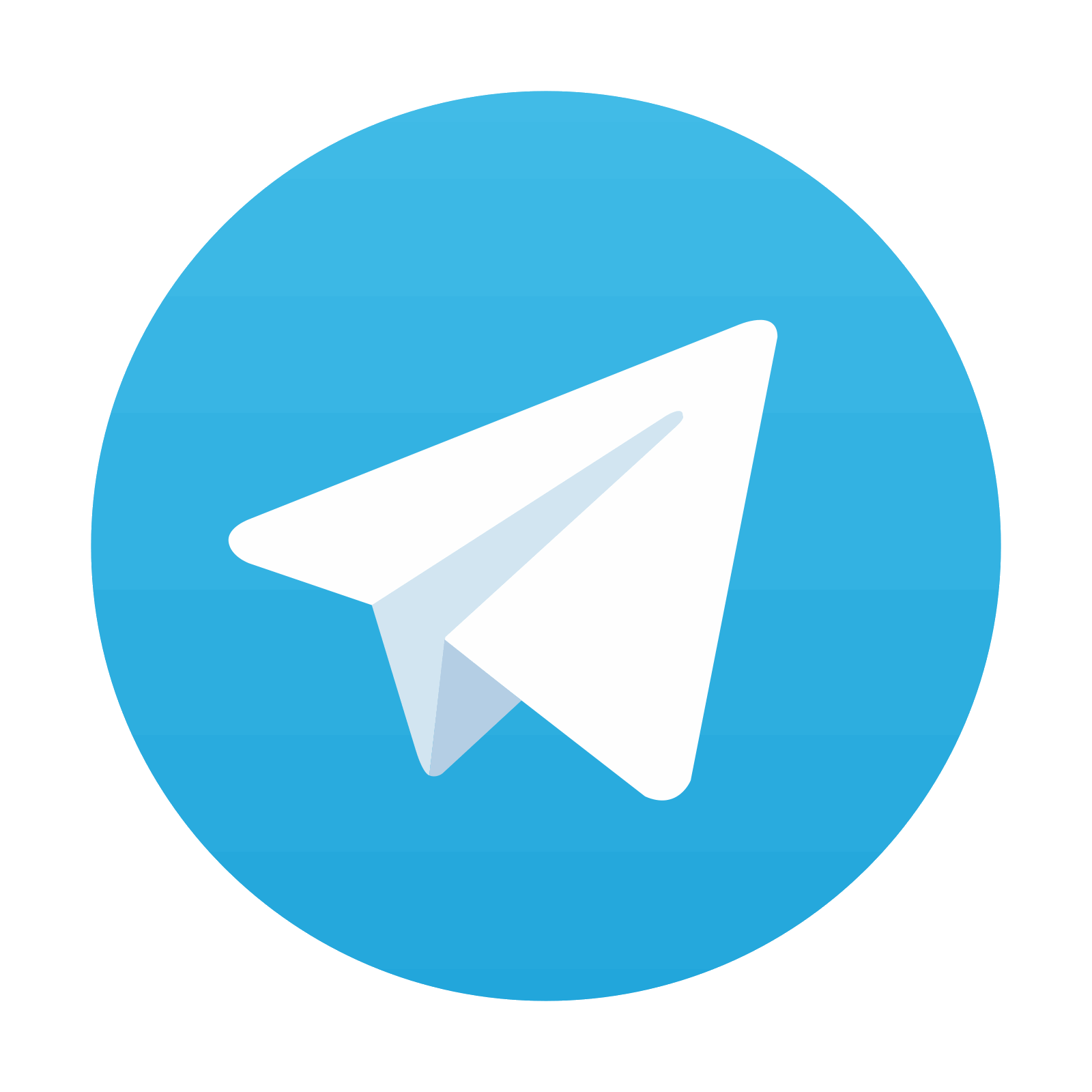
Stay updated, free dental videos. Join our Telegram channel

VIDEdental - Online dental courses
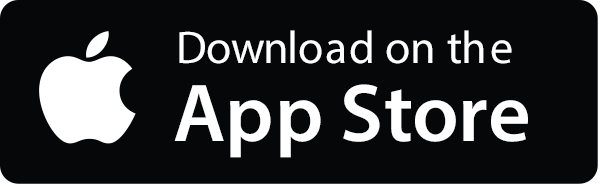
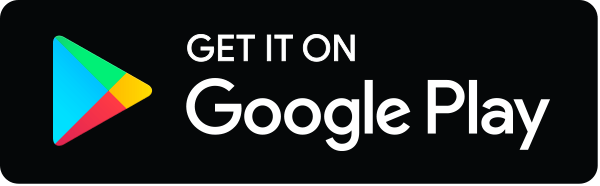
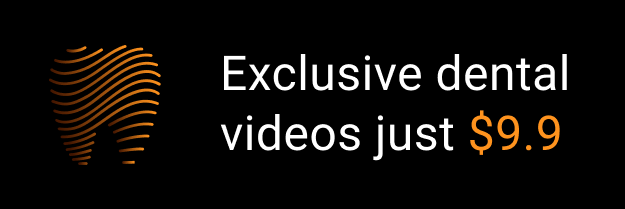