Introduction
The aim was to study nickel-titanium closed-coil springs in a clinically relevant test setting with respect to the accuracy of the “preactivation” for nickel-titanium closed-coil springs application and whether it is possible to keep activation forces constant during the whole time of treatment.
Methods
We tested 10 types of springs from 5 manufacturers under clinically relevant conditions, allowing us to study the interactions between load and temperature over time. Hystereses were compared using t tests.
Results
Springs with a large mechanical hysteresis also showed a large thermal hysteresis. After heating shock, these springs showed intensive force spikes and persistent high loads. Some springs showed negligible thermal and mechanical hysteresis. Such springs never showed any clinically significant persistent high loads.
Conclusions
Springs with a large hysteresis were unable to keep activation forces constant during the whole time of treatment even after any preactivation, and they might cause persistently high loads and possibly overloading. Only springs with minor hysteresis, low temperature dependence of force, and a clinically useful plateau have the following clinical advantages: reduced chair time, optimal rates of tooth movement, reproducible clinical results, and conservation of anchorage.
Highlights
- •
Springs with large hysteresis do not maintain a constant force over time.
- •
The effects of preactivation are cancelled by the intake of warm food.
- •
Springs with minor hysteresis deliver more consistent, predictable forces.
In orthodontics, nickel-titanium (NiTi) materials are mainly used for appliances when a constant force in a wide range of extension is required: eg, archwires and closed-coil springs. Gaps will close faster and more fluently with NiTi springs than with elastic modules. NiTi alloys are distinguished by several uncommon features such as shape-memory effects, superelasticity, and superplasticity. “Superelasticity” refers to their nonelastic stress-strain curves, meaning that a superelastic wire or coil spring will produce a nearly constant force over a large range of deformations called the plateau.
The superelastic properties are connected with phase transformations of the crystallographic structure of the NiTi alloy between a martensitic phase (at lower temperatures softer, behaving similar to tin) and an austenitic phase (at higher temperatures more solid, harder, behaving similar to steel). The transition from the martensitic to the austenitic phase can be induced by the application of a load or stress deformation (so-called mechanoelasticity), a change of temperature (so-called thermoelasticity), or a combination of the two. In orthodontics, the mechanoelasticity of NiTi alloys is typically induced by “activation”: eg, by stretching a closed-coil spring across a gap between 2 teeth.
The transformations from austenite to martensite and back from martensite to austenite do not proceed symmetrically; ie, loading and unloading curves are not identical. The deformation stress necessary for the austenite to martensite transition is higher than the one needed for the martensite to austenite transition under a given strain. This phenomenon is called mechanical hysteresis. It applies also for transformations induced thermoelastically, when it is called thermal hysteresis. Furthermore, one key property of NiTi materials—the working range plateau—can be induced not only by applied stress, but also by temperature changes.
The temperatures of the transition between particular phases are determined by the exact composition, thermal history, and mechanical processing of the NiTi material and can be selected in advance. Many studies have dealt with transformation temperatures of individual material phases and their consequences for practical usage. We can observe finishing temperatures for the martensite transformation from −30°C to −50°C or even lower, as shown in some studies. In medicine, body temperature is used for the transition between the 2 phases. Since NiTi should be in the austenite state to show superelasticity, the finishing temperature for the austenite transformation should be lower than body temperature (37°C), optimally even lower than the common minimum temperatures in patients’ mouths (21°C). However, the oral cavity is thermally a relatively unstable environment. Temperatures in the mouth can vary from 4.3°C (observed in an extreme case) to 65°C. This might be problematic because even minor temperature changes can cause significant changes in the force exerted by a NiTi coil spring.
Barwart compared the dependence of force on temperature in the range from 10°C to 50°C for various NiTi alloys and demonstrated the existence of thermal hysteresis. Other studies have shown that significant increases of transformation temperatures can occur when a NiTi wire is loaded or activated; this further complicates the situation. Vidoni et al studied the impact of thermal and mechanical cycles on material aging, concluding that thermal cycling does not affect the mechanical properties of the springs studied.
The previous statements imply 2 fundamental phenomena. First, mechanical preactivation can be replaced by thermal preactivation. Mechanical preactivation is the process of extending a NiTi closed-coil spring to a length greater than necessary for gripping, whereas thermal preactivation involves supercooling of an already applied spring below the martensite transformation temperature. Second, in case of significant hysteresis, any preactivation is instantly cancelled by ingestion of hot food (eg, soup, coffee, tea). To our knowledge, these 2 fundamental phenomena have not been investigated previously.
The purpose of this investigation was to clarify these 2 fundamental phenomena and draw clinically relevant implications. The key research questions are the following. What is the value of the current recommendation for “preactivation” of NiTi closed-coil spring applications? Is it possible to keep the acting force constant under typically varying temperatures in a patient’s mouth for the whole time of treatment?
Material and methods
For testing, we used a universal testing system (3343; Instron, Canton, Mass) for measuring force and extension. The load frame was equipped with a ±10 N static load cell ( Fig 1 ). To maintain constant conditions of measurement (length and diameter of the spring, formation of bending and torsion forces), measuring appliances of our own construction were terminated by hooks with sharp arches to prevent the springs from moving or rotating against the axis of measurement. A thermostatic water bath with heat exchanger, into which the test coil springs were immersed during measurement, was controlled by a thermostat (f25; Julabo, Allentown, Pa) for setting and keeping the bath at the temperature chosen. The accuracy of the temperature set was ± 0.1°C. A measurement system (VOLTCRAFT IR 1600-50D; Conrad Electronic SE, Hirschau, Germany) with a thermocouple type K recorded the actual bath temperatures over time. The sensor was calibrated at 37°C by comparison with the calibrated sensor of the Julabo f25. The accuracy of the temperature measured was ± 0.2°C.
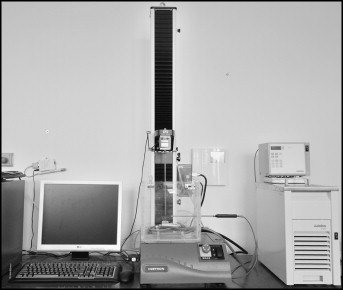
We tested 10 different types of springs from 5 manufacturers (GAC International, Bohemia, NY; 3M Unitek, Monrovia, Calif; Dentaurum, Ispringen, Germany; Ortho Organizers, Carlsbad, Calif; and American Orthodontics, Sheboygan, Wis) ( Table ). For each type of spring, we performed a number of stability and destructive tests to discover the optimal plateau phase deployment and measurement limits for safe spring operation to prevent any unwanted inelastic deformations, eyelet shifts, or even destruction. Five samples of each spring type were tested. To test whether it is possible to keep acting forces of NiTi closed-coil springs constant during the whole time of treatment with or without the help of any preactivation, we chose 2 springs. To achieve the relevant comparison, the 2 springs were of the same group of 9-mm springs. One had the largest mechanical hysteresis of our samples (GAC International, 9 mm Light, 100 g, catalog number, 10-000-03; hereafter called GAC Light); the other had the smallest hysteresis (3M Unitek, 9 mm Medium, 200 g, catalog number, 344-200; hereafter called 3M Medium).
Abbreviation | Manufacturer | Type | Catalog number | Speed of extension change (mm·s −1 ) | Cycles maximum extension (mm) |
---|---|---|---|---|---|
9 mm | |||||
3M 9 | 3M Unitek | Medium 200 g | 344-200 | 2 | 12 |
AO 9 | American Orthodontics | 0.030 in/0.76 mm | 855-180 | 2 | 14 |
DB | Dentaurum | Tomas coil spring light (blue) | 302-012-00 | 2 | 12 |
DY | Dentaurum | Tomas coil spring medium (yellow) | 302-012-10 | 2 | 12 |
GAC L | GAC International | Light (100 g) | 10-000-03 | 2 | 12 |
GAC H | GAC International | Heavy (200 g) | 10-000-01 | 2 | 12 |
OO 9 | Ortho Organizers | 0.010 × 0.030 (200 g) | 100-622 | 2 | 19 |
12 mm | |||||
3M 12 | 3M Unitek | Medium 200 g | 346-200 | 2 | 26 |
AO 12 | American Orthodontics | 0.03 in/0.76 mm | 855-181 | 2 | 26 |
OO 12 | Ortho Organizers | 0.010 × 0.030 (200 g) | 100-623 | 2 | 29 |
To find the appropriate springs—ie, the one with the largest and the one with the smallest mechanical hysteresis—we performed so-called single extension tests (test 1). Then we performed simulations of ingesting warm and cold food (test 2). Finally, to improve the analysis and to deduce the clinically relevant implications, we compared the results from test 2 with test 1.
In test 1a, for multiple comparisons of the load curves and the hystereses of the springs, we performed so-called single-extension tests. At a constant temperature of 37°C, the NiTi closed coil springs were activated to 6 mm extension for the group of 9-mm springs or (test 1b) 12-mm extension for the group of 12-mm springs and then deactivated back to their original spring length. In test 1c, to enhance comparisons of the load curves of the GAC Light and the 3M Medium at constant temperature vs variable temperature, we performed single-extension tests with maximum extensions of 2.6 mm at a constant temperature of 37°C.
In test 2, we performed simulations of ingesting warm food (eg, hot coffee) and cooling back to body temperature in the oral cavity by warming from 37°C to 57°C and back down to 37°C. We also simulated ingesting cold food (eg, a cold drink or ice cream) and warming back to body temperature by cooling from 37°C to 17°C and back up to 37°C. A cycle of warming by 20°C with reversion, or of cooling by 20°C with reversion, took 15 minutes. We chose an extension of 2.6 mm with preactivation of 6 mm because it is a typical clinical situation of closing a gap after extraction of premolars. During the test, we measured and recorded the applied force, the spring extension, and the environment temperature.
In test 2a, at a constant temperature of 37°C, the NiTi closed coil springs were activated to 6 mm extension and then reversed to 2.6 mm extension: ie, to approximately the middle part of the deactivation plateau. Keeping the extension constant at 2.6 mm, temperature cycling began by warming the spring from 37°C to 57°C, then cooling down to 17°C, warming to 57°C, cooling to 17°C, and warming again to 37°C. Finally, at a constant temperature of 37°C, the spring was deactivated back to its original spring length.
In test 2b, at a constant temperature of 37°C, the NiTi closed-coil springs were activated by a 6-mm extension and then reversed to a 2.6-mm extension. Keeping the extension constant at 2.6 mm, a single heating shock was applied by warming the spring from 37°C to 57°C and cooling it back down to 37°C. Finally, at a constant temperature of 37°C, the spring was deactivated back to its original spring length.
Statistical analysis
Measurement data were processed and statistically evaluated with Excel software (version 2007; Microsoft, Redmond, Wash), NCSS 2007 (J. Hintze, Kaysville, Utah; www.ncss.com ), and PASS 2005 (J. Hintze; www.ncss.com ). We used the D’Agostino kurtosis normality test to check the assumption of normality of the distribution. We compared the mechanical hystereses with the 2-sample t test (and the Levene test to check the homoscedasticity assumption). To adjust for multiple comparisons and keep the family-wise α at 0.05, we used the Bonferroni correction. The resulting α for a single comparison was 0.0011.
Results
The data showed considerable intersample variability with respect to mechanical hysteresis ( Fig 2 ). The GAC Light had the highest mechanical hysteresis (40.0 ± 0.7 g), whereas the lowest mechanical hysteresis was found for the 3M Medium (2.4 ± 0.1 g). Of the 9-mm springs, the Ortho Organizers 0.010 × 0.030 (200 g) and the 3M Medium had the lowest mechanical hystereses (4.5 ± 0.1 and 4.8 ± 0.1 g, respectively). There was no statistically significant difference between their mechanical hystereses ( P = 0.002).
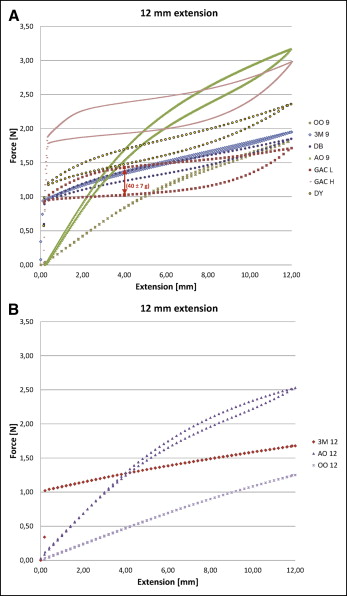
The 3M Medium showed considerably lower thermal hysteresis and force sensitivity in relation to temperature changes when compared with the GAC Light ( Fig 3 ). The thermal hysteresis was negligible for the 3M Medium ( Figs 3 and 4 ). The thermal hysteresis was 8 g (0.08 N) for the GAC Light ( Figs 5 and 6 ).
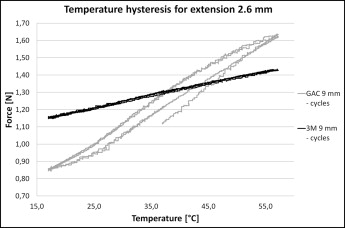
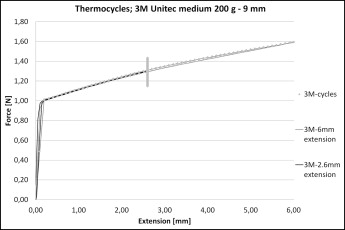
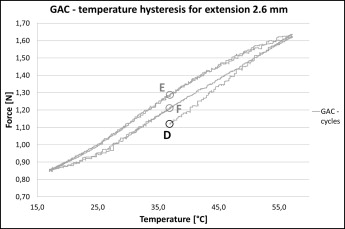
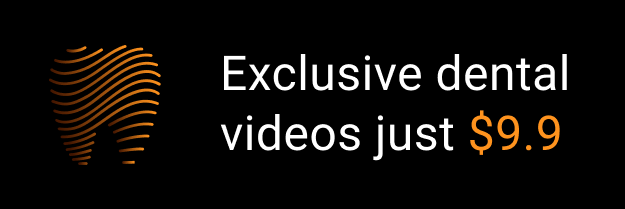