The body fluids
Publisher Summary
This chapter focuses on body fluids. The human body is regarded as a colloidal solution of great complexity as there is a continuous aqueous phase throughout. The obvious differences in composition that occur from one region to another are maintained by physiological barriers that take the form of membranes and intercellular macromolecules. Except in the case of oxygen, entry of material into the body occurs by absorption across the intestinal epithelium, which is subjected to a constantly changing chemical environment. Apart from the great variety of natural food constituents, it may also be exposed to a tremendous range of synthetic compounds devised by food scientists and pharmaceutical chemists. The life of an intestinal epithelial cell is extremely short, and the whole of the intestinal lining is replaced in rather less than 2 days. Although energy-requiring transport mechanisms ensure that valuable materials such as sugars and amino acids are actively absorbed into the bloodstream, useless and even harmful substances may pass across the intestinal barrier if their physicochemical characteristics are such to allow them to traverse it by passive means. Once water and other materials have been absorbed, they are distributed among the various fluid compartments.
Gain | Loss | ||
(ml) | (ml) | ||
Drinks | 1000 | Urine | 1500 |
Solid and semi-solid food | 1200 | Expired air | 350 |
Metabolic water | 300 | Through the skin | 500 |
— | In faeces | 150 | |
2500 | 2500 |
The blood
< ?xml:namespace prefix = "mml" />
The structure and metabolism of haem
Haemoglobin is interesting from many points of view and it has probably been studied more extensively than any other protein. Its shape and general characteristics and its relationship to myoglobin have already been described (page 65) but little has been said about the haem group. Myoglobin, which consists of a single polypeptide chain, has one haem group while haemoglobin, which has four globin subunits, correspondingly has four haem groups, each of which can combine with one molecule of oxygen. Globin itself is colourless and contains a greater proportion of basic amino acids than any of the other blood proteins. Although the haemoglobins of all mammals are similar in shape and structure, they show appreciable differences in their amino acid composition, affinity for O2 and other gases, and in their crystalline form. All these differences are attributable to the globin since the haem group is the same in all species. Haem is an iron–porphyrin compound.
placed iron atom is bound to the four N atoms of the pyrrole rings leaving two of the six coordination valencies on the iron atom free. In both myoglobin and haemoglobin the iron atom of the haem is directly bonded to a histidine residue. This is known as the proximal histidine. Oxygen binding occurs at the sixth coordination position near which the second important or distal histidine is situated. In deoxyhaemoglobin this position is empty.
Bile pigments
When, at the end of their life span, for unknown reasons the red cells rupture, the haemoglobin is released. It first combines with haptoglobins (page 379) present in the plasma which have a special affinity for Hb and, while combined in this way, the iron is oxidized to the ferric form. Either before or after combination with haptoglobins the haemoglobin is taken up by cells of the reticuloendothelial system, mainly in the liver, spleen and bone marrow. Here it is split into its constituent parts which are independently processed. The globin is broken down to amino acids which pass into the metabolic pool while one of the methine bridges of the porphyrin ring is oxidized, the iron is released and a chain-like tetrapyrrole compound is produced. The first bile pigment to be formed is biliverdin which is green. This is readily reduced to bilirubin which is golden brown and the chief pigment of human bile. The bilirubin is transported from the reticuloendothelial cells to the liver in combination with serum albumin. In the liver it is conjugated with glucuronic acid and the conjugated bilirubin, which is water-soluble, is excreted in bile into the intestine.
which is colourless, but this is subsequently oxidized to orange–yellow stercobilin (urobilin) which contributes to the normal colour of the faeces.
The functions of haemoglobin and myoglobin
In the haemoglobin molecule, although the four haem groups are separated by appreciable distances, the state of one haem is influenced by that of the other three and these haem–haem interactions are of considerable physiological importance. This can be judged by comparing the properties of haemoglobin and myoglobin. As can be seen from Figure 25.1, the O2 dissociation curve of myoglobin is a simple rectangular hyperbola while that of haemoglobin is sigmoid. The two pigments are well suited for their respective physiological roles. Thus myoglobin acts as a store of O2 in muscles that may be deprived of O2 for relatively long periods. For this purpose it is important that it should take up O2 when it is readily available and release it only when it cannot be obtained from the atmosphere. From the curve it can be seen that at an O2 pressure of 20 mmHg myoglobin is 80% saturated with O2 and only releases significant amounts at O2 pressures below this. Haemoglobin on the other hand, although it also has storage capacity for O2, is essentially a carrier and is required alternately to pick up and release O2 with changes of O2 pressure operating at a higher range.
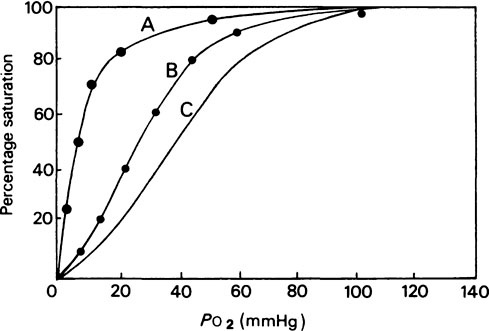
The difference in properties between the two proteins, whose tertiary structures are very similar (page 67), is due to the superimposed quaternary structure of haemoglobin and the fact that the ease with which any haem group binds O2 is determined by the state of the other three. Starting with deoxyhaemoglobin the first O2 molecule is taken up very slowly, the second and third are taken up more and more readily and the fourth is taken up several hundred times more rapidly than the first; hence the sigmoid shape of the curve.
Changes in haemoglobin in response to the environment
In deoxyhaemoglobin the iron atom lies about 0·6 Å (0·06 nm) out of the plane of the ring but, on oxygenation, it moves into the plane of the ring and is able to form a strong bond with oxygen (Figure 25.2). This tiny structural change within the subunits is translated into a shift in the relationship between them. One pair of αβ subunits rotates relatively to the other pair causing the β chains to move closer together and the α chains to move slightly away from one another. This change in the quaternary structure on oxygenation and deoxygenation may be regarded as a ‘breathing movement’ at the molecular level.
Not only does haemoglobin have multiple binding sites for oxygen which, as described above, show positive cooperativity, it also combines at different sites with CO2 and H+ ions and also with the substance 2,3-diphosphoglycerate, and a complex relationship exists between the binding of these various ligands. This is physiologically highly advantageous and has the result that when oxygenated blood from the lungs reaches the tissues where H+ and CO2 are being produced, the combined effects of the lower O2 tension and higher H+ and CO2 concentrations ensure that O2 is released in appropriate amounts while, at the same time, CO2 is taken up. The promotion of O2 dissociation by an increase in CO2 tension and therefore of acidity is known as the Bohr Effect and is illustrated in Figure 25.1.
The role of haemoglobin in carbon dioxide transport
Haemoglobin plays a part in the carriage of CO2 from the tissues to the lungs in two ways:
1. It combines directly with the CO2 which reacts with free amino groups to form carbamino compounds.

2. It has a buffering effect. When haemoglobin, which is notably rich in histidine, is oxygenated the resulting conformational changes increase the tendency for specific protonated histidine residues to lose H+, i.e. their pK value in oxyhaemoglobin is 7·16 compared with 7·3 in deoxyhaemoglobin. Thus, in the lungs, the proportion of HbO2 is increased and that of HHb is reduced. Conversely, in the tissues, deoxyhaemoglobin binds H+; at the same time the plasma bicarbonate is increased (see the next paragraph). In this way a large proportion of the acid produced in the course of the tissue metabolism is carried as H+ by haemoglobin in the corpuscles and as bicarbonate in the plasma.
The overall process of CO2 uptake and O2 release can be represented as follows:
The role of the plasma bicarbonate in acid–base regulation
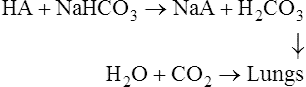
The carbonic acid which is produced causes a fall in the NaHCO3/H2CO3 ratio and a slight drop in the pH of the blood. The overall effect is to stimulate the respiratory centre of the brain causing an increase in pulmonary ventilation so that the extra CO2 is lost from the lungs and the normal NaHCO3/H2CO3 ratio is restored. However, in the course of the reaction some of the plasma bicarbonate has been used up so that, although the NaHCO3/H2CO3 ratio and pH are normal, the plasma bicarbonate is reduced. The responsibility for regulating the absolute amount of bicarbonate in the plasma belongs to the kidney (page 395).
Plasma proteins
For clinical investigations of the plasma proteins serum is commonly used instead of plasma since fibrinogen and fibrin tend to interfere with some of the tests. The serum proteins are usually separated on a variety of materials when five distinct fractions are produced. These are albumin and the α1, α2, β and γ globulin fractions. Typical electrophoretic patterns for normal serum are shown in Figure 25.3 and the percentage composition of the total plasma proteins in Table 25.1.
Table 25.1
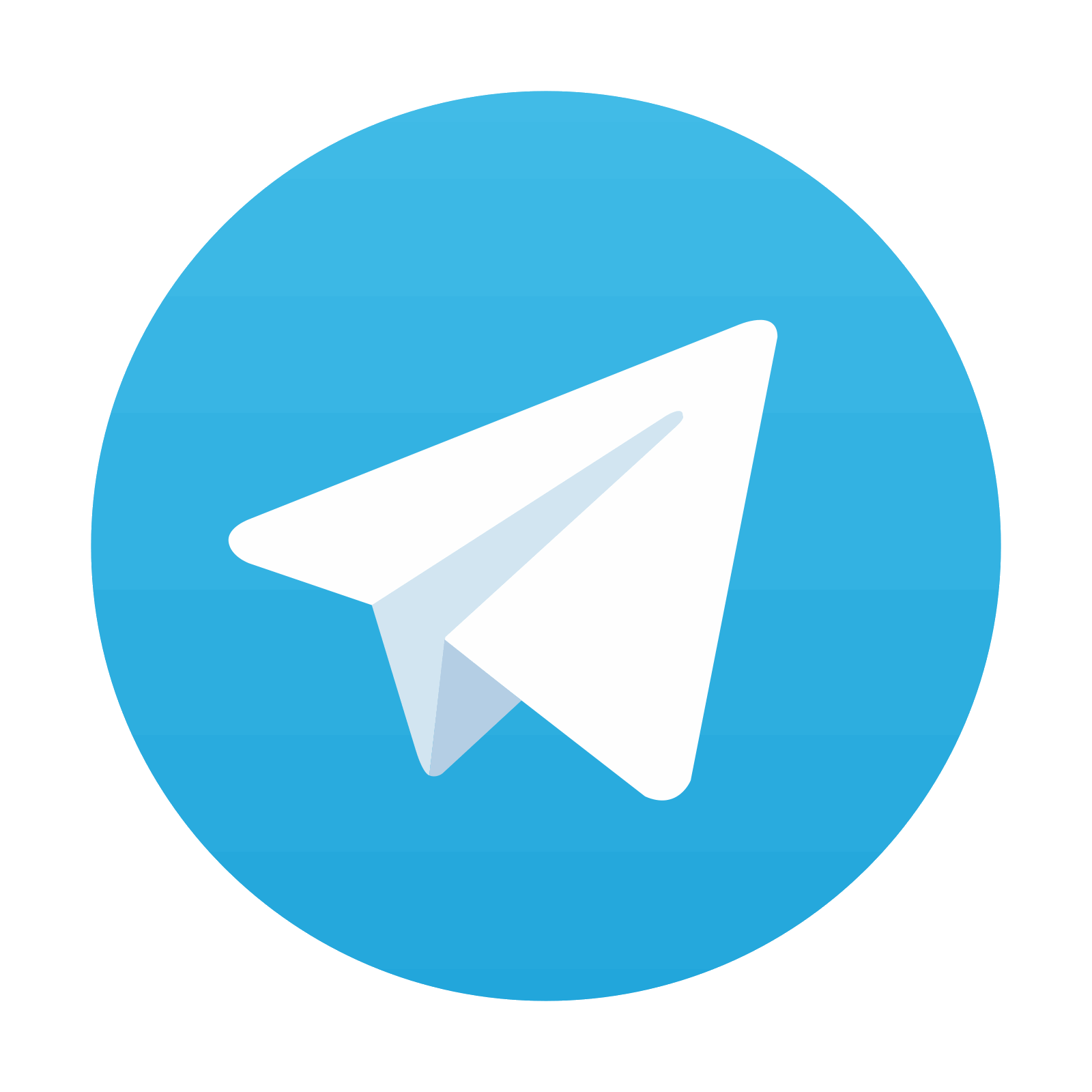
Stay updated, free dental videos. Join our Telegram channel

VIDEdental - Online dental courses
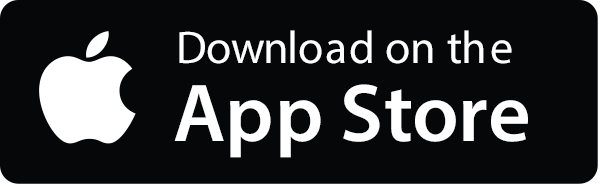
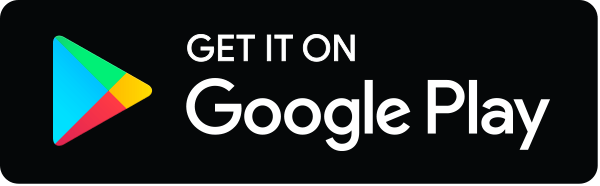