1 The authors acknowledge the outstanding work of Drs. Henry Trowbridge, Syngcuk Kim, Hideaki Suda, David H. Pashley, and Fredrick R. Liewehr in previous editions of this text. The present chapter is built on their foundational work.
Morphologic Zones of the Pulp
The Pulp-Dentin Complex
The dental pulp and dentin function as a unit, and the odontoblasts represent a crucial element in this system. The odontoblasts are located in the periphery of the pulp tissue, with extensions into the inner part of dentin. Dentin would not exist unless produced by odontoblasts, and the dental pulp is dependent on the protection provided by the dentin and enamel. Likewise, integrated dynamics of the pulp-dentin complex imply that impacts on dentin may affect the pulpal components and that disturbances in the dental pulp will in turn affect the quantity and quality of the dentin produced.
Odontoblast Layer
The outermost stratum of cells of the healthy pulp is the odontoblast layer ( Figs. 12-1 and 12-2 ). This layer is located immediately subjacent to the predentin. The odontoblast processes, however, pass on through the predentin into the inner part of dentin. Consequently, the odontoblast layer is actually composed of the cell bodies of odontoblasts. In addition, capillaries, nerve fibers, and dendritic cells may be found among the odontoblasts.


In the coronal portion of a young pulp that is actively secreting collagen, the odontoblasts assume a tall columnar form. The odontoblasts vary in height; consequently, their nuclei are not all at the same level and are aligned in a staggered array, often described as a palisade appearance. This organization makes the layers appear to be three to five cells in thickness even though there is only one actual layer of odontoblasts. Between adjacent odontoblasts there are small intercellular spaces approximately 30 to 40 nm in width. Odontoblast cell bodies are connected by tight and gap junctional complexes. Gap junctions are formed by connexin proteins that permit cell-to-cell passage of signal molecules.
The odontoblast layer in the coronal pulp contains more cells per unit area than in the radicular pulp. Whereas the odontoblasts of the mature coronal pulp are usually columnar, those in the midportion of the radicular pulp are more cuboidal ( Fig. 12-3 ). Near the apical foramen, the odontoblasts appear as a squamous layer of flattened cells. Because fewer dentinal tubules per unit area are present in the root than in the crown of the tooth, the odontoblast cell bodies are less crowded and are able to spread out laterally. During maturation and aging, there is a continued ongoing crowding in the odontoblast layer, particularly in the coronal pulp, due to narrowing of the pulp space. Apoptosis of odontoblasts seems to adjust for this limited space during development.

There is a series of specialized cell-to-cell junctions (i.e., junctional complexes), including desmosomes (i.e., zonula adherens), gap junctions (i.e., nexuses), and tight junctions (i.e., zonula occludens) that connect adjacent odontoblasts. Spot desmosomes located in the apical part of odontoblast cell bodies mechanically join odontoblasts together. The numerous gap junctions provide permeable pathways through which signal molecules can pass between cells ( Fig. 12-4 ) to synchronize secretory activity that produces relatively uniform predentin layers (see Fig. 12-2 ). These junctions are most numerous during the formation of primary dentin. Gap junctions and desmosomes have also been observed joining odontoblasts to the processes of fibroblasts in the subodontoblastic area. Tight junctions are found mainly in the apical part of odontoblasts in young teeth. These structures consist of linear ridges and grooves that close off the intercellular space. However, tracer studies suggest direct passage of small elements from subodontoblastic capillaries to predentin and dentin between the odontoblasts. It appears that tight junctions determine the permeability of the odontoblast layer when dentin is covered by enamel or cementum by restricting the passage of molecules, ions, and fluid between the extracellular compartments of the pulp and predentin. During cavity preparation, these junctions are disrupted, thereby increasing dentin permeability.

Cell-Poor Zone
Immediately subjacent to the odontoblast layer in the coronal pulp, there is often a narrow zone approximately 40 µm in width that is relatively free of cells (see Fig. 12-1 ) and hence is called the cell-free layer of Weil . It is traversed by blood capillaries, unmyelinated nerve fibers and the slender cytoplasmic processes of fibroblasts (see Fig. 12-2 ). The presence or absence of the cell-poor zone depends on the functional status of the pulp. It may not be apparent in young pulps, where dentin forms rapidly, or in older pulps, where reparative dentin is being produced.
Cell-Rich Zone
In the subodontoblastic area, there is a stratum containing a relatively high proportion of fibroblasts compared with the more central region of the pulp (see Fig. 12-1 ). It is much more prominent in the coronal pulp than in the radicular pulp. Besides fibroblasts, the cell-rich zone may include a variable number of immune cells like macrophages and dendritic cells, but also undifferentiated mesenchymal stem cells.
On the basis of evidence obtained in rat molar teeth, it has been suggested that the cell-rich zone forms as a result of peripheral migration of cells populating the central regions of the pulp, commencing at about the time of tooth eruption. Migration of immunocompetent cells out of and into the cell-rich zone has been demonstrated as a result of antigenic challenge. Although cell division within the cell-rich zone is rare in normal pulps, the death of the odontoblasts triggers a great increase in the rate of mitosis. Because odontoblasts are postmitotic cells, irreversibly injured odontoblasts are replaced by cells that migrate from the cell-rich zone onto the inner surface of the dentin. This mitotic activity is probably the first step in the formation of a new odontoblast layer. Studies implicate stem cells as a source for these replacement odontoblasts.
Pulp Proper
The pulp proper is the central mass of the pulp (see Fig. 12-1 ). It consists of loose connective tissue and contains the larger blood vessels and nerves. The most prominent cell in this zone is the fibroblast.
Cells of the Pulp
Odontoblast
Because odontoblasts are responsible for dentinogenesis, both during tooth development and aging, the odontoblast is the most characteristic and specialized cell of the dentin-pulp complex. During dentinogenesis, the odontoblasts form dentin and the dentinal tubules, and their presence within the tubules makes dentin a living responsive tissue.
Dentinogenesis, osteogenesis, and cementogenesis are in many respects quite similar, and odontoblasts, osteoblasts, and cementoblasts have many characteristics in common. Each of these cells produces a matrix composed of collagen fibrils, noncollagenous proteins, and proteoglycans that are capable of undergoing mineralization. The ultrastructural characteristics of odontoblasts, osteoblasts, and cementoblasts are likewise similar in that each exhibits a highly ordered rough endoplasmic reticulum (RER), a prominent Golgi complex, secretory granules, and numerous mitochondria. In addition, these cells are rich in RNA, and their nuclei contain one or more prominent nucleoli. These are the general characteristics of protein-secreting cells.
The most significant differences among odontoblasts, osteoblasts, and cementoblasts are their morphologic characteristics and the anatomic relationship between the cells and the mineralized structures they produce. Whereas osteoblasts and cementoblasts are polygonal to cuboidal in form, the fully developed odontoblast of the coronal pulp is a tall columnar cell. In bone and cementum, some of the osteoblasts and cementoblasts become entrapped in the matrix as osteocytes or cementocytes, respectively. The odontoblast, on the other hand, leaves behind a cellular process to form the dentinal tubule, and the cell body resides outside the mineralized tissue. Lateral branches between the major odontoblast processes interconnect through canaliculi, just as osteocytes and cementocytes are linked through the canaliculi in bone and cementum. This provides a pathway for intercellular communication and circulation of fluid and metabolites through the mineralized matrix.
The ultrastructural features of the odontoblast have been the subject of numerous investigations. The cell body of the active odontoblast has a large nucleus that may contain up to four nucleoli ( Fig. 12-5 ). The nucleus is situated at the basal end of the cell and is contained within a nuclear envelope. A well-developed Golgi complex, centrally located in the supranuclear cytoplasm, consists of an assembly of smooth-walled vesicles and cisternae. Numerous mitochondria are evenly distributed throughout the cell body. RER is particularly prominent, consisting of closely stacked cisternae forming parallel arrays that are dispersed diffusely within the cytoplasm. Numerous ribosomes closely associated with the membranes of the cisternae mark the sites of protein synthesis. Within the lumen of the cisternae, filamentous material (probably representing newly synthesized protein) can be observed.

The odontoblast appears to synthesize mainly type I collagen, although small amounts of type V collagen have been found in the extracellular matrix (ECM). In addition to proteoglycans and collagen, the odontoblast secretes dentin sialoprotein and phosphophoryn, a highly phosphorylated phosphoprotein involved in extracellular mineralization. Phosphophoryn is unique to dentin and is not found in any other mesenchymal cell types. The odontoblast also secretes both acid phosphatase and alkaline phosphatase. The latter enzyme is closely linked to mineralization, but the precise role of alkaline phosphatase in dentinogenesis is not completely understood. Acid phosphatase, a lysosomal enzyme, may be involved in digesting material that has been resorbed from predentin matrix.
In contrast to the active odontoblast, the resting or inactive odontoblast has a decreased number of organelles and may become progressively shorter. These changes can begin with the completion of root development and eruption when dentin production shifts from primary to secondary dentin.
Direct actions of odontoblasts on dental nerves and vice versa have been proposed based on the excitability of odontoblasts, the differential expression of receptors for neuropeptides on odontoblasts ( Fig. 12-6 ), the demonstration of the thermosensitive transient receptor potential (TRP) ion channels, and the finding that all nine voltage-gated sodium channels are variably expressed on odontoblasts in developing, mature, and aging rat teeth. In addition, a possible function of odontoblast in immune regulation has been proposed by the finding of innate immune components in the odontoblast layer. Hence, the odontoblasts should be capable of recognizing and differentially responding to bacterial components, thereby serving immune and pulp-dentine barrier functions.

Odontoblast Process
A dentinal tubule forms around each of the major odontoblastic processes. The odontoblast process occupies most of the space within the tubule and coordinates the formation of peritubular dentin.
Microtubules and microfilaments are the principal ultrastructural components of the odontoblast process and its lateral branches. Microtubules extend from the cell body out into the process. These straight structures follow a course that is parallel with the long axis of the cell and impart the impression of rigidity. Although their precise role is unknown, theories as to their functional significance suggest that they may be involved in cytoplasmic extension, transport of materials, or the provision of a structural framework. Occasionally, mitochondria can be found in the process where it passes through the predentin.
The plasma membrane of the odontoblast process closely approximates the wall of the dentinal tubule. Localized constrictions in the process occasionally produce relatively large spaces between the tubule wall and the process. Such spaces may contain collagen fibrils and fine granular material that presumably represents ground substance (see also The Pulpal Interstitium and Ground Substance in this chapter). The peritubular dentin matrix within the tubule is lined by an electron-dense limiting membrane called the lamina limitans . A narrow space separates the limiting membrane from the plasma membrane of the odontoblast process, except for the areas where the process is constricted.
In restoring a tooth, the removal of enamel and dentin often disrupts odontoblasts. It would be of considerable clinical importance to establish the extent of the odontoblast processes in human teeth, because with this knowledge, the clinician would be in a better position to estimate the impact of the restorative procedure on the underlying odontoblasts. However, the extent to which the process penetrates into dentin has been a matter of considerable controversy. It has long been thought that the process is present throughout the full thickness of dentin. Although ultrastructural studies using transmission electron microscopy have described the process as being limited to the inner third of the dentin, it should be noted that this could possibly be the result of shrinkage occurring during fixation and dehydration. Other studies employing scanning electron microscopy have described the process extending further into the tubule, often as far as the dentoenamel junction (DEJ), but it has been suggested that what has been observed in scanning electron micrographs is actually the lamina limitans.
In an attempt to resolve this issue, monoclonal antibodies directed against microtubules were used to demonstrate tubulin in the microtubules of the process. Immunoreactivity was observed throughout the dentinal tubule, suggesting that the process extends throughout the thickness of dentin. However, a study employing confocal microscopy found that odontoblast processes in rat molars do not extend to the outer dentin or DEJ, except during the early stages of tooth development. It is likely that the walls of tubules contain many proteins originally derived from odontoblasts that no longer remain at that site. Because dentin matrix has no turnover, these antigens remain fixed in place. From a clinical perspective, it is important to remember that these processes in the tubules represent appendages from living odontoblasts in the pulp, which explains why the dentin must be considered a vital tissue, the destruction of which will affect the pulp.
The odontoblast is considered to be a fixed postmitotic cell in that once it has fully differentiated, it apparently cannot undergo further cell division. If this is indeed the case, the life span of the odontoblast coincides with the life span of the viable pulp. However, its metabolic activity can be dynamically altered (described under the heading Pulpal Repair).
Relationship of Odontoblast Structure to Secretory Function
Studies using radiolabeled chemicals have shed a great deal of light on the functional significance of the cytoplasmic organelles of the active odontoblast. In experimental animals, intraperitoneal injection of a collagen precursor (e.g., 3 H-proline) is followed by autoradiographic labeling of the odontoblasts and predentin matrix ( Fig. 12-7 ). Rapid incorporation of the isotope in the RER soon leads to labeling of the Golgi complex in the area where the procollagen is packed and concentrated into secretory vesicles. Radiolabeled vesicles can then be followed along their migration pathway until they reach the base of the odontoblast process. Here they fuse with the cell membrane and release their tropocollagen molecules into the predentin matrix by the process of exocytosis.

It is now known that collagen fibrils precipitate from a solution of secreted tropocollagen and that the aggregation of fibrils occurs on the outer surface of the odontoblast plasma membrane. Fibrils are released into the predentin and increase in thickness as they approach the mineralized matrix. Whereas fibrils at the base of the odontoblast process are approximately 15 nm in diameter, fibrils in the region of the calcification front have attained a diameter of about 50 nm.
Similar tracer studies have elucidated the pathway of synthesis, transport, and secretion of the predentin proteoglycans. The protein moiety of these molecules is synthesized by the RER of the odontoblast, whereas sulfation and addition of the glycosaminoglycan (GAG) moieties to the protein molecules take place in the Golgi complex. Secretory vesicles then transport the proteoglycans to the base of the odontoblast process, where they are secreted into the predentin matrix. Proteoglycans, principally chondroitin sulfate, accumulate near the calcification front. The role of the proteoglycans is speculative, but mounting evidence suggests that they act as inhibitors of calcification by binding calcium. It appears that just before calcification, the proteoglycans are removed, probably by lysosomal enzymes secreted by the odontoblasts.
Pulp Fibroblast
Fibroblasts are the most numerous cells of the pulp. They appear to be tissue-specific cells capable of giving rise to cells that are committed to differentiation (e.g., odontoblast-like cells) if given the proper signal. These cells synthesize types I and III collagen, as well as proteoglycans and GAGs. Thus, they produce and maintain the matrix proteins of the ECM. Because they are also able to phagocytose and digest collagen, fibroblasts are responsible for collagen turnover in the pulp.
Although distributed throughout the pulp, fibroblasts are particularly abundant in the cell-rich zone. The early differentiating fibroblasts are polygonal and appear to be widely separated and evenly distributed within the ground substance. Cell-to-cell contacts are established between the multiple processes that extend out from each of the cells. Many of these contacts take the form of gap junctions that provide for electronic coupling or chemical signaling from one cell to another. In terms of ultrastructure, the organelles of the immature fibroblasts are generally in a rudimentary stage of development, with an inconspicuous Golgi complex, numerous free ribosomes, and sparse RER. As they mature, the cells become stellate in form, and the Golgi complex enlarges, the RER proliferates, secretory vesicles appear, and the fibroblasts take on the characteristic appearance of protein-secreting cells. In addition, collagen fibrils accumulate along the outer surface of the cell body. With an increase in the number of blood vessels, nerves, and collagen fibers, there is a relative decrease in the number of fibroblasts in the pulp.
Many fibroblasts of the pulp are characterized by being relatively undifferentiated. A more modern term for undifferentiated cells is stem cells . Many pulpal cells do seem to remain in a relatively undifferentiated modality, compared with fibroblasts of most other connective tissues. This perception has been supported by the observation of large numbers of reticulin-like fibers in the pulp. Reticulin fibers have an affinity for silver stains and are similar to the argyrophilic fibers of the pulp. However, in a careful review, it appears that actual reticulin fibers may not be present in the pulp; instead the previously described fibers are actually argyrophilic collagen fibers . The fibers apparently acquire a GAG sheath, and it is this sheath that is impregnated by silver stains. In the young pulp, the nonargyrophilic collagen fibers are sparse, but they progressively increase in number as the pulp ages.
Many experimental models have been developed to study wound healing in the pulp, particularly dentinal bridge formation after pulp exposure or pulpotomy. One study demonstrated that mitotic activity preceding the differentiation of replacement odontoblasts appears to occur primarily among perivascular fibroblasts.
Pulpal fibroblasts seem to take active part in signaling pathways in the dental pulp. For example, fibroblast growth and synthesis are stimulated by neuropeptides; in turn, fibroblasts produce nerve growth factor (NGF) and proinflammatory cytokines during inflammation. NGF plays an important role not only in tooth development but also in regulating neuronal and possibly odontoblast responses to injury via activation of similar neurotrophin receptors expressed on both cell types (see also Plasticity of Intradental Nerve Fibers in this chapter).
Macrophage
Macrophages are monocytes that have left the bloodstream, entered the tissues, and differentiated into various subpopulations. The different subpopulations can be studied by their antigenic properties in immunohistochemical studies. Many are found in close proximity to blood vessels. A major subpopulation of macrophages is active in endocytosis and phagocytosis ( Fig. 12-8 ). Because of their mobility and phagocytic activity, they are able to act as scavengers, removing extravasated red blood cells, dead cells, and foreign bodies from the tissue. Ingested material is destroyed by the action of lysosomal enzymes. Another subset of macrophages participates in immune reactions by processing antigen and presenting it to memory T cells. The processed antigen is bound to class II major histocompatibility complex (MHC) molecules on the macrophage, where it can interact with specific receptors present on naive or memory T cells. Such interactions are essential for T cell–dependent immunity. Similar to fibroblasts, macrophages take an active part in the signaling pathways in the pulp. When activated by the appropriate inflammatory stimuli, macrophages are capable of producing a large variety of soluble factors, including interleukin 1, tumor necrosis factor, growth factors, and other cytokines. One study showed that a subset of macrophages express lymphatic markers, indicating a link between macrophages and lymphatic function and development.

Dendritic Cell
Dendritic cells are accessory cells of the immune system. Similar cells are found in the epidermis and mucous membranes, where they are called Langerhans cells . Dendritic cells are primarily found in lymphoid tissues, but they are also widely distributed in connective tissues, including the pulp ( Fig. 12-9 ). These cells are termed antigen-presenting cells and are characterized by dendritic cytoplasmic processes and the presence of class II MHC complexes on their cell surface ( Fig. 12-10 ). In the normal pulp they are mostly located in the periphery of the coronal pulp close to the predentin, but they migrate centrally in the pulp after antigenic challenge. They are known to play a central role in the induction of T cell–dependent immunity. Like antigen-presenting macrophages, dendritic cells engulf protein antigens and then present an assembly of peptide fragments of the antigens and MHC class II molecules. It is this assembly that T cells can recognize. Then the assembly binds to a T-cell receptor and T-cell activation occurs ( Fig. 12-11 ). Fig. 12-12 shows a cell-to-cell contact between a dendritic-like cell and a lymphocyte.




Lymphocyte
Hahn and colleagues reported finding T lymphocytes in normal pulps from human teeth. T8 (suppressor) lymphocytes were the predominant T-lymphocyte subset present in these samples. Lymphocytes have also been observed in the pulps of impacted teeth. The presence of macrophages, dendritic cells, and T lymphocytes indicates that the pulp is well equipped with cells required for the initiation of immune responses. B lymphocytes are scarcely found in the normal uninflamed pulp.
Mast Cell
Mast cells are widely distributed in connective tissues, where they occur in small groups in relation to blood vessels. Mast cells are seldom found in the normal pulp tissue, although they are routinely found in chronically inflamed pulps. The mast cell has been the subject of considerable attention because of its dramatic role in inflammatory reactions. The granules of mast cells contain heparin, an anticoagulant, and histamine, an important inflammatory mediator, as well as many other chemical factors.
Metabolism
The metabolic activity of the pulp has been studied by measuring the rate of oxygen consumption and the production of carbon dioxide or lactic acid. An investigation employed an oxygen-sensitive microelectrode inserted into a rat incisor pulp with a micromanipulator. The authors reported that odontoblasts consumed O 2 at the rate of 3.2 ± 0.2 ml/min/100 g of pulp tissue.
Because of the relatively sparse cellular composition of the pulp, the rate of oxygen consumption is low in comparison with that of most other tissues. During active dentinogenesis, metabolic activity is much higher than after the crown is completed. As would be anticipated, the greatest metabolic activity is found in the region of the odontoblast layer, and the lowest is found in the central pulp, where most of the nerves and blood vessels are located.
In addition to the usual glycolytic pathway, the pulp has the ability to produce energy through a phosphogluconate (i.e., pentose phosphate) shunt type of carbohydrate metabolism, a metabolic pathway that permits tissues to function under varying degrees of ischemia. This could explain how the pulp manages to withstand periods of low perfusion resulting from vasoconstriction induced by epinephrine-containing infiltration anesthesia.
Several commonly used dental materials (e.g., eugenol, zinc oxide and eugenol, calcium hydroxide, silver amalgam) inhibit oxygen consumption by pulp tissue, indicating that these agents may be capable of depressing the metabolic activity of pulpal cells. One study found that application of orthodontic force to human premolars for 3 days resulted in a 27% reduction in respiratory activity in the pulp. This study utilized carbon-14-labeled succinic acid in the medium. As cells metabolize succinic acid, they produce CO 2 that can be trapped and quantitated by a liquid scintillation counter. This technique requires only a few milligrams of tissue.
The Pulpal Interstitium and Ground Substance
The interstitium consists of the interstitial fluid and the interstitial (extracellular) matrix and occupies the extracellular and extravascular space. It is amorphous and generally regarded as a gel rather than a solid. Its constituents are similar in all tissues, but their relative amount varies. The major structural component of the interstitium is collagen ( Fig. 12-13 ). The network of collagen fibers also supports the other components of the interstitium, the proteoglycans, hyaluronan, and elastic fibers. The two former components represent the glycosaminoglycans of the interstitial matrix.

Because of its content of polyanionic polysaccharides, the interstitium is responsible for the water-holding properties of connective tissues and acts as a molecular sieve in regulating the diffusion of substances through this space. The magnitude of the excluded volume has important consequences because the effective protein concentration in the interstitium is higher than the value that would be estimated from fluid volume per se.
Connective tissue consists of cells and fibers, both embedded in ground substance or ECM. Cells that produce connective tissue fibers also synthesize the major constituents of the ECM. Whereas the fibers and cells have recognizable shapes, the ECM is described as being amorphous. It is generally regarded as a gel rather than a solid. Because of its content of polyanionic polysaccharides, the ECM is responsible for the water-holding properties of connective tissues.
Nearly all proteins of the ECM are glycoproteins. Proteoglycans are an important subclass of glycoproteins. These molecules support cells, provide tissue turgor, and mediate a variety of cell interactions. They have in common the presence of GAG chains and a protein core to which the chains are linked. Except for heparan sulfate and heparin, the chains are composed of disaccharides. The primary function of GAG chains is to act as adhesive molecules that can bond to cell surfaces and other matrix molecules.
Fibronectin is a major surface glycoprotein that, together with collagen, forms an integrated fibrillary network that influences adhesion, motility, growth, and differentiation of cells. Laminin, an important component of basement membranes, binds to type IV collagen and cell surface receptors. Tenascin is another substrate adhesion glycoprotein.
In the pulp, the principal proteoglycans include hyaluronic acid dermatan sulfate, heparan sulfate, and chondroitin sulfate. The proteoglycan content of pulp tissue decreases approximately 50% with tooth eruption. During active dentinogenesis, chondroitin sulfate is the principal proteoglycan, particularly in the odontoblast and predentin layer, where it is somehow involved with mineralization; with tooth eruption, hyaluronic acid and dermatan sulfate increase, and chondroitin sulfate decreases greatly.
The consistency of a connective tissue (e.g., the pulp) is largely determined by the proteoglycan components of the ground substance. The long GAG chains of the proteoglycan molecules form relatively rigid coils constituting a network that holds water, thus forming a characteristic gel. Hyaluronic acid in particular has a strong affinity for water and is a major component of ground substance in tissues with a large fluid content, such as Wharton’s jelly of the umbilical cord. The water content of the young pulp is very high (approximately 90%), so the ground substance forms a cushion capable of protecting cells and vascular components of the tooth.
Ground substance also acts as a molecular sieve in that it excludes large proteins. Cell metabolites, nutrients, and wastes pass through the ground substance between cells and blood vessels. In some ways, ground substance can be likened to an ion exchange resin, because the polyanionic chains of the GAGs bind cations. In addition, osmotic pressures can be altered by excluding osmotically active molecules. Thus, proteoglycans can regulate the dispersion of interstitial matrix solutes, colloids, and water, and (in large measure) they determine the physical characteristics of a tissue, such as the pulp.
Degradation of ground substance can occur in certain inflammatory lesions that have a high concentration of macrophage lysosomal enzymes. Proteolytic enzymes, hyaluronidases, and chondroitin sulfatases of lysosomal and bacterial origin are examples of the hydrolytic enzymes that can attack components of the ground substance. The pathways of inflammation and infection are strongly influenced by the state of polymerization of the ground substance components.
Hyaluronan
Another major structural component of the interstitial matrix is hyaluronan. It is an unbranched, random-coil molecule from repeating nonsulfated disaccharide units and is found in the interstitium as free molecules or bound to cells, possibly via the connection to fibronectin. Its large molecular weight together with its protein structure accounts for its unique properties. It has a high viscosity even at low concentration, exhibits exclusion properties, and has a strong affinity for water.
Hyaluronan is one of several types of GAGs in the pulp. The hyaluronan receptor-1 is expressed on lymphatic vessels and also on immune cells in the dental pulp. Hyaluronan is removed from the tissue by the lymphatics and metabolized in the lymph nodes and by endothelial cells in the liver.
Elastic Fibers
Elastic fibers constitute an elastin core and a surrounding microfibrillar network and provide elasticity to the tissue. The amount of elastin in the interstitial matrix in most tissue is small. There is no evidence for elastic fibers in the matrix in the pulp.
The Inflamed Interstitium
Hyaluronidases, and chondroitin sulfatases of lysosomal and bacterial origin, are examples of the hydrolytic enzymes that can attack components of the interstitium. During infection and inflammation, the physical properties of the pulp tissue may then be altered due to production of such degrading enzymes. In addition to their own damaging effect, they may also pave the way for the deleterious effects of bacterial toxins, increasing the magnitude of the damage.
The pathways of inflammation and infection are strongly influenced by the particular composition of the interstitium in every tissue and its degradation by either host or microbial enzymes.
Connective Tissue Fibers of the Pulp
Two types of structural proteins are found in the pulp: collagen and elastin. Elastin fibers are confined to the walls of arterioles and, unlike collagen, are not a part of the ECM.
A single collagen molecule, referred to as tropocollagen, consists of three polypeptide chains, designated as either alpha-1 or alpha-2 depending on their amino acid composition and sequence. In the human pulp, the amount of collagen is reported to be 26% to 32% of dry weight in premolars and molars. Type I and type III collagen represent the major subtypes of collagen in the pulp, and type I is found in thick striated fibrils throughout the pulp tissue. The different combinations and linkages of chains making up the tropocollagen molecule have allowed collagen fibers and fibrils to be classified into a number of types:
- ◆
Type I collagen is found in skin, tendon, bone, dentin, and pulp.
- ◆
Type II collagen is found in cartilage.
- ◆
Type III collagen is found in most unmineralized connective tissues. It is a fetal form found in the dental papilla and the mature pulp. In bovine pulp, it constitutes 45% of the total pulp collagen during all stages of development.
- ◆
Types IV and VII collagen are components of basement membranes.
- ◆
Type V collagen is a constituent of interstitial tissues.
- ◆
Type VI collagen is a heterotrimer of three distinct chains, alpha 2 (VI) and alpha 3 (VI), and is widely distributed in low concentrations in soft tissues at interfibrillar filaments.
Type I collagen is synthesized by odontoblasts and osteoblasts; fibroblasts synthesize types I, III, V, and VII collagen.
In collagen synthesis, the protein portion of the molecule is formed by the polyribosomes of the RER of connective tissue cells. The proline and lysine residues of the polypeptide chains are hydroxylated in the cisternae of the RER, and the chains are assembled into a triple-helix configuration in the smooth endoplasmic reticulum. The product of this assembly is termed procollagen, and it has a terminal unit of amino acids known as the telopeptide of the procollagen molecule . When these molecules reach the Golgi complex, they are glycosylated and packaged in secretory vesicles. The vesicles are transported to the plasma membrane and secreted by way of exocytosis into the extracellular milieu, thus releasing the procollagen. Here the terminal telopeptide is cleaved by a hydrolytic enzyme, and the tropocollagen molecules begin aggregating to form collagen fibrils. It is believed that the GAGs somehow mediate aggregation of tropocollagen. The conversion of soluble collagen into insoluble fibers occurs as a result of cross-linking of tropocollagen molecules. The presence of collagen fibers passing from the dentin matrix between odontoblasts into the dental pulp has been reported in fully erupted teeth. Larger collagen fiber bundles are much more numerous in the radicular pulp than in the coronal pulp. The highest concentration of these larger fiber bundles is usually found near the apex ( Fig. 12-14 ). Pulpectomy procedures should engage the pulp with a barbed broach in the region of the apex, as it generally affords the best opportunity to remove this tissue intact.

The Trigeminal System
Innervation
Pain is a subjective phenomenon involving not only sensory physiologic responses, but also emotional, conceptual, and motivational aspects of behavior. The existence of peripheral “nociceptive” (pain-detecting) sensory neurons forms the basis for pain, and pain sensations of varying qualities and intensities are evoked by activation of the intradental nerves innervating teeth. Noxious stimuli in teeth are transmitted in primary afferent neurons located in the trigeminal ganglion via second-order neurons in the brain stem to the brain ( Fig. 12-15 ; see also Chapter 17 and later in this chapter). Transmission of sensory information consists of a cascade of events involving input, processing, and sensing, so the control of dental pain should be based on an understanding of the origin of pain signals and the complex modulation that may take place locally and at higher levels. The sensory system of the pulp appears to be well suited for signaling potential damage to the tooth. The tooth is innervated by a large number of myelinated and unmyelinated axons. The number of axons entering a human premolar may reach 2000 or more, and each axon can arborize to form multiple points of innervation.

Regardless of the nature of the sensory stimulus (i.e., thermal, mechanical, chemical, electric [e.g., pulp tester]), almost all afferent impulses generated from pulp tissue result in the sensation of pain. However, when the pulp is weakly stimulated by an electric pulp tester under carefully controlled experimental conditions, a nonpainful sensation (i.e., prepain) has been reported. Thus, not all afferent neurons that innervate the pulp are nociceptors. The innervation of the pulp includes both afferent neurons, which conduct sensory impulses, and autonomic or efferent neurons, which provide neurogenic modulation of the microcirculation, inflammatory reactions, and perhaps regulate dentinogenesis.
The sympathetic innervation of teeth derives from the superior cervical ganglion (SCG). Postganglionic sympathetic nerves travel with the internal carotid nerve, join the trigeminal nerve at the ganglion, and supply teeth and supporting structures via the maxillary and mandibular division of the trigeminal nerve. Sympathetic fibers appear with blood vessels at the time the vascular system is established in the dental papilla. In the adult tooth pulp, sympathetic fibers form plexuses, usually around pulpal arterioles ( Fig. 12-16 ). Stimulation of these fibers results in constriction of the arterioles and a decrease in blood flow. The sympathetic neuron terminals contain the classic neurotransmitter, norepinephrine (NE), and neuropeptide Y (NPY) (see Neuropeptides later in this chapter). NPY is synthesized in sympathetic neurons and supplied to terminals by axonal transport. By contrast, NE is mainly produced locally in the terminals. Compared with the sensory nerves, these fibers are most often located in deeper parts of the pulp proper, but fibers have also been found in close relation to odontoblasts.

The presence of parasympathetic cholinergic nerves in dental tissues has been and is still controversial, although it has been concluded that there is absence of parasympathetic vasodilation in the cat dental pulp. It has been reported that the neuropeptide vasoactive intestinal polypeptide (VIP) is localized in the parasympathetic neurons. The origin of VIP-containing fibers in the pulp is uncertain insofar as no form of surgical denervation has resulted in complete loss of these fibers from the dental pulp.
Sensory nerve fibers are usually classified according to their diameter, conduction velocity, and function as shown in Table 12-1 . The pulp contains two types of sensory nerve fibers: myelinated (A fibers) and unmyelinated (C fibers). It has been shown that there is some functional overlap between pulpal A and C fibers, as both fiber types can be nociceptors. The A fibers include both A-beta and A-delta fibers. The A-beta fibers may be slightly more sensitive to stimulation than the A-delta fibers, but functionally these fibers are grouped together in the dental pulp, because both innervate the dentinal tubules, and both are stimulated by dentinal fluid movement ( Fig. 12-17 ). Approximately 90% of the A fibers in dental pulp are A-delta fibers. Table 12-2 summarizes the principal characteristics of the main sensory fibers.
Type of Fiber | Function | Diameter (µm) | Conduction Velocity (m/sec) |
---|---|---|---|
A-alpha | Motor, proprioception | 12-20 | 70-120 |
A-beta | Pressure, touch | 5-12 | 30-70 |
A-gamma | Motor, to muscle spindles | 3-6 | 15-30 |
A-delta | Pain, temperature, touch | 1-5 | 6-30 |
B | Preganglionic autonomic | < 3 | 3-15 |
C dorsal root | Pain | 0.4-1 | 0.5-2 |
Sympathetic | Postganglionic sympathetic | 0.3-1.3 | 0.7-2.3 |

Fiber | Myelination | Location of Terminals | Pain Characteristics | Stimulation Threshold |
---|---|---|---|---|
A-delta | Yes | Principally in region of pulp-dentin junction | Sharp, pricking | Relatively low |
C | No | Probably distributed throughout pulp | Burning, aching, less bearable than A-delta fiber sensations | Relatively high, usually associated with tissue injury |
During the bell stage of tooth development, “pioneer” nerve fibers enter the dental papilla following the path of blood vessels. Although only unmyelinated fibers are observed in the dental papilla, a proportion of these fibers are probably A fibers that have lost or not developed their myelin sheath. Myelinated fibers are the last major structures to appear in the developing human dental pulp. The number of nerve fibers gradually increases, and branching occurs as the fibers approach dentin. During the bell stage, very few fibers enter the predentin.
The sensory nerves of the pulp arise from the trigeminal nerve and pass into the radicular pulp in bundles by way of the foramen in close association with arterioles and venules ( Fig. 12-18 ). Each of the nerves entering the pulp is invested within Schwann cells, and the A fibers acquire their myelin sheath from these cells. With the completion of root development, the myelinated fibers appear grouped in bundles in the central region of the pulp ( Fig. 12-19 ). Most of the unmyelinated C fibers entering the pulp are located within these fiber bundles; the remainder is situated toward the periphery of the pulp (see Fig. 12-17 ). It should be noted that single neurons have been reported to innervate the pulps of multiple teeth in animal studies. Assuming a similar innervation pattern in humans, this finding may partially explain why patients often have difficulty localizing pulpal pain to a specific tooth. An alternative explanation to this clinical observation is that the pulp has a relatively low density of proprioceptors, and thus patients have difficulty identifying the inflamed tooth until the inflammation reaches the periradicular tissue, which is highly innervated with proprioceptors. This is discussed in greater detail in Chapter 17 .


In the human premolar, the number of unmyelinated axons entering the tooth at the apex reached a maximal number shortly after tooth eruption. At this stage, an average of 1800 unmyelinated axons and more than 400 myelinated axons were found, although in some teeth fewer than 100 myelinated axons were present. Five years after eruption, the number of A fibers gradually increased to more than 700. The relatively late appearance of A fibers in the pulp may help to explain why the electric pulp test tends to be unreliable in young teeth, as A fibers are more easily electrically stimulated than C fibers.
A quantitative study of axons 1 to 2 mm coronal to the root apex of fully developed human canine and incisor teeth reported a mean of about 360 myelinated axons in canines and incisors, whereas there were 1600 to 2200 unmyelinated axons. However, this does not reflect the actual number of neurons supporting a single tooth, because multiple branching of the axons may occur in the peripheral tissues. Overall, approximately 80% of the axons were unmyelinated fibers.
The nerve bundles pass upward through the radicular pulp together with blood vessels (see Fig. 12-18 ). Once they reach the coronal pulp, they fan out beneath the cell-rich zone, branch into smaller bundles, and finally ramify into a plexus of single-nerve axons known as the plexus of Raschkow ( Fig. 12-20 ). Full development of this plexus does not occur until the final stages of root formation. It has been estimated that each axon entering the pulp sends at least eight branches to the plexus of Raschkow. There is prolific branching of the fibers in the plexus, producing a tremendous overlap of receptor fields. It is in the plexus that the A fibers emerge from their encircling Schwann cells and branch repeatedly to form the subodontoblastic plexus. Finally, terminal axons pass between the odontoblasts as free nerve endings ( Figs. 12-21 and 12-22 ). The extent to which dentin is innervated has been the subject of numerous investigations. With the exception of the innervation of dentinal tubules, discussed later in this chapter, the bulk of dentin is devoid of sensory nerve fibers. This offers an explanation as to why pain-producing agents (e.g., potassium chloride) do not always elicit pain when applied to exposed dentin. Similarly, application of topical anesthetic solutions to dentin does not decrease its sensitivity. A high concentration of lidocaine solution is needed to block the response of intradental nerves to mechanical stimulation of the dentin.



One investigator studied the distribution and organization of nerve fibers in the dentin-pulp border zone of human teeth. On the basis of their location and pattern of branching, several types of nerve endings were described ( Fig. 12-23 ). Some fibers were found running from the subodontoblastic nerve plexus toward the odontoblast layer. However, these fibers do not reach the predentin; they terminate in extracellular spaces in the cell-rich zone, the cell-poor zone, or the odontoblast layer. Other fibers extend into the predentin and run through a dentinal tubule in close association with an odontoblast process. Most of these intratubular fibers extend into the dentinal tubules for only a few micrometers, but a few may penetrate as far as 100 µm (see Fig. 12-20 ). The area covered by a single such terminal complex often reaches thousands of square micrometers.

Intratubular nerve endings are most numerous in the area of the pulp horns, where as many as 40% of the tubules may contain fibers. The number of intratubular fibers decreases in other parts of the dentin, and in root dentin only about 1% of dentinal tubules contain fibers. This notion has been challenged in a study that stained pulps for protein gene-product 9.5, a specific marker for nerves. In that study, root dentin appeared to be as well innervated as coronal dentin. The anatomic relationship between the odontoblast processes and sensory nerve endings has led to much speculation as to the functional relationships between these structures, if any. When present, nerve fibers lie in a groove or gutter along the surface of the odontoblast process, and toward their terminal ends, they twist around the process like a corkscrew. The cell membranes of the odontoblast process and the nerve fiber are closely approximated and run closely parallel for the length of their proximity, but they are not synaptically linked.
Although it may be tempting to speculate that the odontoblasts and their associated nerve axons are functionally interrelated and that together they play a role in dentin sensitivity, there is a paucity of evidence supporting this hypothesis. If the odontoblast were acting as a classic receptor cell, * it would have chemical, electric, or mechanical communication with the adjacent nerve fiber. However, researchers have been unable to find classical anatomic structures (e.g., synaptic junctions) that could functionally couple odontoblasts and nerve fibers together. With regard to the membrane properties of odontoblasts, it has been reported that the membrane potential of the odontoblast is low (−24 to −30 mV), and that the cell does not respond to electric stimulation. It would appear that the odontoblast does not possess the properties of an excitable cell. Further, the sensitivity of dentin is not diminished after disruption of the odontoblast layer. It is still possible that odontoblasts could modulate neuronal function via alteration in sodium channel activity or the release of paracrine factors that diffuse to the closely approximated nerve terminal.
* A receptor cell is a non–nerve cell capable of exciting adjacent afferent nerve fibers. Synaptic junctions connect receptor cells to afferent nerves.
Another study showed that a reduction in pulpal blood flow, induced by stimulation of sympathetic fibers leading to the pulp, results in depressed excitability of pulpal A fibers. The excitability of C fibers is less affected than that of A fibers by a reduction in blood flow.
Of clinical interest is the evidence that nerve fibers of the pulp may be resistant to necrosis because their cell bodies are found in ganglia outside the pulp. Because nerve bundles in general are more resistant to autolysis than other tissue elements, even in degenerating pulps, C fibers might still be able to respond to noxious stimulation. It may be that C fibers remain excitable even after blood flow has been compromised in the diseased pulp, as C fibers are often able to function in the presence of hypoxia. This may explain why instrumentation of the root canals of apparently nonvital teeth sometimes elicits pain. On the other hand, histologic studies on nonvital teeth failed to demonstrate high levels of innervation, leading to the suggestion that pain may be due to the transfer of noxious chemicals to terminals located in periapical tissues.
Steps and Mechanisms in Pain Perception
When activated by a stimulus sufficient to cause tissue damage or release of inflammatory mediators, nerve endings in the pulp and periradicular tissues begin to send bursts of messages to the central nervous system (CNS) that may eventually be perceived as pain. The anatomic pathway for this transmission of information has been fairly well established, and it is tempting to view the perception of pain of orofacial origin as a simple graded response to the intensity of the stimulus. However, researchers have come to realize that the pain system is a complex, multilevel system that begins with the detection of tissue-damaging stimuli in the periphery, the processing of that input at the level of the medullary spinal cord, and the perception of what is felt as pain in higher brain regions such as the cerebral cortex ( Fig. 12-24 ). After a noxious stimulus is detected in the periphery, there is ample opportunity for a great deal of endogenous and possibly exogenous modification of the message prior to its ultimate perception. The clinician deals with all three levels of the pain system in diagnosing and treating odontalgia, and a practitioner with a basic understanding of each level will be able to recognize therapeutic opportunities and apply effective pain control methods.

Detection: The First Step in Pain Perception
Various types of peripheral neurons are found in the trigeminal system, including large-diameter, heavily myelinated Aα, Aβ, and Aγ fibers associated with motor, proprioception, touch, pressure, and muscle spindle stretch functions. But it is the smaller, less myelinated Aδ and yet smaller and unmyelinated C fibers that conduct information likely to be perceived as pain. These two classes of pain-sensing nerve fibers, or nociceptors, are both found in the tooth pulp, but there are three to eight times more unmyelinated C fibers than Aδ fibers. It should be noted that this classification system is based purely on the size and myelination of the neurons and does not necessarily indicate function. For example, another class of pulpal C fibers are the postganglionic sympathetic efferents found in association with blood vessels, where they regulate pulpal blood flow and may also influence the activity of peripheral nociceptors (for reviews, see Perl or Hargreaves ). Because most pulpal sensory fibers are nociceptive, their terminal branches are free nerve endings, and physiologic stimulation by any modality (temperature, hyperosmotic fluids) results in the perception of pure pain, which can be difficult for patients to localize. Under experimental conditions, electrical stimulation can result in a prepain sensation that is also difficult to localize. Once inflammation has extended to the periodontal ligament, which is well endowed with Aβ discriminative touch receptors, localization of pain is more predictable with light mechanical stimuli such as the percussion test.
In the normal uninflamed pulp and periradicular tissues, a noxious stimulus causes depolarization of nociceptors sufficient to generate action potentials by means of the opening of voltage-gated sodium channels (Na v ). After generation of an action potential, not only is information sent to the CNS, but also in an antidromic fashion (i.e., in the reverse direction of the impulse) in which proinflammatory neuropeptides such as substance P (SP), calcitonin gene-related peptide (CGRP), neurokinins, and the classic neurotransmitter, glutamate, are released from afferent terminals in the pulp and periradicular tissues.
Neuropeptides
Of immense importance in pulp biology is the presence of neuropeptides in pulpal nerves. Pulpal nerve fibers contain neuropeptides such as calcitonin gene–related peptide (CGRP) (see Figs. 12-18, 12-20, and 12-21 ), substance P (SP), neuropeptide Y (see Fig. 12-16 ), neurokinin A (NKA), and VIP. In rat molars, the largest group of intradental sensory fibers contains CGRP. Some of these fibers also contain other peptides, such as SP and NKA. Release of these peptides can be triggered by numerous stimuli, including tissue injury, complement activation, antigen-antibody reactions, or antidromic stimulation of the inferior alveolar nerve. Once released, vasoactive peptides produce vascular changes that are similar to those evoked by histamine and bradykinin (i.e., vasodilation). In addition to their neurovascular properties, SP and CGRP contribute to inflammation and promote wound healing. The release of CGRP can be modified by sympathetic agonists and antagonists, offering the promise of using such agonists to treat dental pain. This latter point is important because clinicians use sympathetic agonists every day—the vasoconstrictors present in local anesthetic solutions may have direct effects on inhibiting dental nerve activity. Local anesthetic reduction in pain may be due to the actions of both the local anesthetic and the vasoconstrictor. In cats, capsaicin acutely activates and chronically blocks the TRPV1-expressing classes of C and A-delta nociceptors in the pulp. In addition, the chronic application of capsaicin ointment to skin has been shown to relieve pain in patients, suggesting that clinical trials evaluating chronic application of capsaicin for treating pulpal or periradicular pain may be of value.
Antidromic stimulation of nerves (i.e., toward the peripheral terminals) simply means that the afferent barrage goes in the opposite direction of the orthograde stimulation (toward the CNS). Normally, sensory nerves are stimulated at their peripheral terminations, and their action potentials then travel toward the brain. In antidromic nerve stimulation, the sensory nerve is usually cut. The peripheral end of the nerve is then electrically stimulated. This causes an action potential to travel backward toward the periphery, which causes release of neuropeptides in the pulp. All branches of the nerve also depolarize and release neuropeptides (the so-called axon reflex).
It has been reported that mechanical stimulation of dentin produces vasodilation within the pulp, presumably by causing the release of neuropeptides from intradental sensory fibers (neurogenic inflammation). Electric stimulation of the tooth has a similar effect. The pulpal concentrations of CGRP, SP, and NKA are elevated in painful human teeth over healthy control teeth extracted for orthodontic reasons. These peptides are also elevated in pulps beneath advancing carious lesions.
Pulp Testing
The electric pulp tester delivers a current sufficient to overcome the resistance of enamel and dentin and stimulate the sensory A fibers at the dentin-pulp border zone. Smaller C fibers of the pulp do not respond to the conventional pulp tester because significantly more current is needed to stimulate them. Bender and associates found that in anterior teeth, the optimal placement site of the electrode is the incisal edge, as the response threshold is lowest at that location and increases as the electrode is moved toward the cervical region of the tooth.
Cold tests using carbon dioxide (CO 2 ) snow or liquid refrigerants and heat tests employing heated gutta-percha or hot water activate hydrodynamic forces within the dentinal tubules, which in turn excite the intradental A fibers. C fibers are generally not activated by these tests unless they produce injury to the pulp. It has been shown that cold tests do not injure the pulp. Heat tests have a greater potential to produce injury, but if the tests are used properly, injury is not likely.
Sensitivity of Dentin
The mechanisms underlying dentin sensitivity have been a subject of interest for many years. How are stimuli relayed from the peripheral dentin to the sensory terminals located in the region of the dentin-pulp border zone? Converging evidence indicates that movement of fluid in the dentinal tubules is the basic event in the arousal of dentinal pain. It now appears that pain-producing stimuli, such as heat, cold, air blasts, and probing with the tip of an explorer, have in common the ability to displace fluid in the tubules. This is referred to as the hydrodynamic mechanism of dentin sensitivity . The hydrodynamic theory suggests that dentinal pain associated with stimulation of a sensitive tooth ultimately involves mechanotransduction. Classical mechanotransducers have been recognized on pulpal afferents, providing a mechanistic support to this theory. Thus, fluid movement in the dentinal tubules is translated into electric signals by receptors located in the axon terminals innervating dentinal tubules. Using single-fiber recording techniques, a positive correlation was found between the degree of pressure change and the number of nerve impulses leaving the pulp ( Figs. 12-25 and 12-26 ). Thus, the outward fluid movements (negative pressure) produce a much stronger nerve response than inward movements.


In experiments on humans, brief application of heat or cold to the outer surface of premolar teeth evoked a painful response before the heat or cold could have produced temperature changes capable of activating sensory receptors in the underlying pulp. The evoked pain was of short duration: 1 or 2 seconds. The thermal diffusivity of dentin is relatively low, yet the response of the tooth to thermal stimulation is rapid, often less than 1 second. Evidence suggests that thermal stimulation of the tooth results in a rapid movement of fluid into the dentinal tubules. This results in activation of the sensory nerve terminal in the underlying pulp. Presumably, heat expands the fluid within the tubules faster than it expands dentin, causing the fluid to flow toward the pulp, whereas cold causes the fluid to contract more rapidly than dentin, producing an outward flow. It is speculated that the rapid movement of fluid across the cell membrane of the axon terminal activates a mechanosensitive receptor, similar to how fluid movement activates hair cells in the cochlea of the ear. All axon terminals have membrane channels through which charged ions pass, and this initial receptor current, if sufficient, can trigger voltage-gated sodium channels to depolarize the cell, leading to a barrage of impulses to the brain. Some ion channels are activated by voltage, some by chemicals, and some by mechanical pressure. In the case of pulpal nerve fibers that are activated by hydrodynamic forces, pressure would be transduced, gating mechanosensitive ion channels.
The dentinal tubule is a capillary tube with an exceedingly small diameter. * The physical properties of capillarity are significant because fluid force increases as the diameter is reduced. If fluid is removed from the outer end of exposed dentinal tubules by dehydrating the dentinal surface with an air blast or absorbent paper, capillary forces produce a rapid outward movement of fluid in the tubule ( Fig. 12-27 ). According to Brännström, desiccation of dentin can theoretically cause dentinal fluid to flow outward at a rate of 2 to 3 mm/sec. In addition to air blasts, dehydrating solutions containing hyperosmotic concentrations of sucrose or calcium chloride can produce pain if applied to exposed dentin.

* To appreciate fully the dimensions of dentin tubules, understand that the diameter of the tubules (about 1 µm) is much smaller than that of red blood cells (about 7 µm). The thickness of coronal dentin is about 3 mm, so each tubule is 3000 µm long but only 1 µm in diameter. Thus, each tubule is 3000 diameters long.
Investigators have shown that it is the A fibers rather than the C fibers that are activated by hydrodynamic stimuli (e.g., heat, cold, air blasts) applied to exposed dentin. However, if heat is applied long enough to increase the temperature of the dentin-pulp border by several degrees Celsius, then C fibers may respond, particularly if the heat produces injury. It seems that the A fibers are mainly activated by a rapid displacement of the tubular contents. Slow heating of the tooth produced no response until the temperature reached 111° F (43.8° C), at which time C fibers were activated, presumably because of heat-induced injury to the pulp. These C fibers are called polymodal nociceptors because they contain numerous receptors that confer the ability to detect and respond to many different types of stimuli. Capsaicin, the pungent active ingredient in hot peppers, is known to simulate C fibers and a subset of A-delta fibers. Capsaicin activates a receptor termed the “transient receptor potential, subtype vanilloid 1” or TRPV1. The TRPV1 receptor is expressed primarily on a major subclass of nociceptors and responds to heat (> 110° F [> 43° C]), certain inflammatory mediators, and acid (pH < 6). Thus TRPV1 has been considered a molecular integrator of polymodal noxious stimuli. The ability of the TRPV1 antagonist, capsazepine, to inhibit acid-, heat-, and capsaicin-activated trigeminal neurons has led to the development of new drugs (i.e., TRPV1 antagonists) for the treatment of pulpal pain. Eugenol is known to activate and ultimately desensitize TRPV1, and this may explain the anodyne action of zinc oxide eugenol temporary restorations.
It has also been shown that pain-producing stimuli are more readily transmitted from the dentin surface when the exposed tubule apertures are open and the fluid within the tubules is free to flow outward. For example, acid treatment of exposed dentin to remove the smear layer opens the tubule orifices and makes the dentin much more responsive to stimuli such as air blasts and probing.
Perhaps the most difficult phenomenon to explain is dentinal pain associated with light probing of dentin. Even light pressure of an explorer tip can produce strong forces. * These forces have been shown to mechanically compress dentin and close open tubule orifices with a smear layer that causes sufficient displacement of fluid to excite the sensory receptors in the underlying pulp ( Fig. 12-28 ). Considering the density of the tubules in which hydrodynamic forces would be generated by probing, multiple nerve endings would be simultaneously stimulated when a dental explorer is scratched across dentin. Another newly suggested explanation is that the nerves innervating teeth are special in nature and that low-threshold mechanoreceptors are signaling nociceptive input in teeth. Similar low-threshold mechanoreceptors convey tactile sensation in skin. The authors used the term low-threshold “algoneurons” for these nerves. This theory is not in conflict with the hydrodynamic theory but may help to explain the sensation of pain felt after weak mechanical stimuli like air puffs and water spray.

* A force of 44 cM (44 g) applied to an explorer having a tip 40 µm in diameter would produce a pressure of 2437 MPa on the dentin. This is far in excess of the compressive strength of dentin, listed as 245MPa, as evidenced by the shallow grooves lined by smear layers created in dentin using this force.
Another example of the effect of strong hydraulic forces that are created within the dentinal tubules is the phenomenon of odontoblast displacement. In this reaction, the nuclei and cell bodies of odontoblasts are displaced upward in the dentinal tubules, presumably by a rapid movement of fluid in the tubules produced when exposed dentin is desiccated, as with the use of an air syringe or cavity-drying agents ( Fig. 12-29 ). Such cellular displacement results in the destruction of odontoblasts, because cells thus affected soon undergo autolysis and disappear from the tubules. Displaced odontoblasts may eventually be replaced by stem cells that migrate from the cell-rich zone of the pulp, as discussed later in the chapter.

The hydrodynamic theory can also be applied to an understanding of the mechanism responsible for hypersensitive dentin. There is controversy regarding whether exposed dentin is simply sensitive or becomes truly hypersensitive. Growing evidence indicates that new sodium channels responsible for activating nerves are expressed in nerve tissue exposed to inflammation. An increase in the density of sodium channels or their sensitivity may contribute to dentinal hypersensitivity. Hypersensitive dentin is also associated with the exposure of dentin normally covered by cementum or enamel. The thin layer of cementum is frequently lost as gingival recession exposes cementum to the oral environment. Cementum is subsequently worn away by brushing, flossing, or using toothpicks. Once exposed, the dentin may respond to the same stimuli to which any exposed dentin surface responds (e.g., mechanical pressure, dehydrating agents). Although the dentin may at first be very sensitive, within a few weeks the sensitivity usually subsides. This desensitization is thought to occur as a result of gradual occlusion of the tubules by mineral deposits, thus reducing hydrodynamic forces. In addition, deposition of reparative dentin over the pulpal ends of the exposed tubules probably also reduces sensitivity, because reparative dentin is less innervated by sensory nerve fibers. However, some hypersensitive dentin does not spontaneously desensitize, so hypersensitivity may be caused by either inflammatory changes in the pulp or mechanical changes in the patency of dentinal tubules.
Currently, the treatment of hypersensitive teeth is directed toward reducing the functional diameter of the dentinal tubules to limit fluid movement. Four possible treatment modalities can accomplish this objective:
- 1.
Formation of a smear layer on the sensitive dentin by burnishing the exposed root surface
- 2.
Application of agents, such as oxalate compounds, that form insoluble precipitates within the tubules
- 3.
Application of agents such as hydroxyethyl methacrylate (HEMA) with or without glutaraldehyde that are thought to occlude tubules with precipitated plasma proteins in dentinal fluid
- 4.
Application of dentin bonding agents to seal off the tubules
Dentin sensitivity can be modified by laser irradiation, but clinicians must be concerned about its effect on the pulp.
Peripheral Sensitization
Following repeated noxious stimuli, both A and polymodal C fiber nociceptors undergo a process of sensitization manifested by three obvious changes in response patterns. First, firing thresholds may decrease, so that previously non-noxious stimuli may trigger discharges, contributing to the sensation of pain (allodynia). Second, after-discharges may occur, so that noxious stimuli may produce an even greater increase in the perceived intensity of pain (hyperalgesia). And third, firing may occur spontaneously, contributing to the development of spontaneous pain. These changes are often seen in endodontic pain patients and may be explained in part by the effects of chemical mediators released into inflamed pulp and periradicular tissues. Such mediators include substances produced from damaged tissues, agents of vascular origin, and peptides released from the nerve fibers themselves ( Table 12-3 ). Other mechanisms of peripheral sensitization are listed in Box 12-1 .
Mediator | Effect on Nociceptors | Effect on Human Volunteers |
---|---|---|
Potassium | Activate | ++ |
Protons | Activate | ++ |
Serotonin | Activate | ++ |
Bradykinin | Activate | +++ |
Histamine | Activate | + |
Tumor necrosis factor-α | Activate | ? |
Prostaglandins | Sensitize | ± |
Leukotrienes | Sensitize | ± |
Nerve growth factor | Sensitize | ++ |
Substance P | Sensitize | ± |
Interleukin 1 | Sensitize (?) | ? |
Mechanism
- ♦
Composition and concentration of inflammatory mediators
- ♦
Changes in afferent fiber: activation and sensitization
- ♦
Changes in afferent fiber: sprouting
- ♦
Changes in afferent fiber: proteins
- ♦
Tissue pressure
- ♦
Tissue temperature
- ♦
Sympathetic primary afferent fiber interactions
- ♦
Aβ fiber plasticity
Hyperalgesia and Allodynia
Three characteristics of hyperalgesia are (1) spontaneous pain, (2) a decreased pain threshold, and (3) an increased response to a painful stimulus. The peripheral mechanisms for these symptoms include a decrease in firing threshold, an increase in responsiveness to noxious stimuli, and development of spontaneous discharges of nociceptors. All three of these characteristics can be seen in patients experiencing inflammatory pain of pulpal origin ( Table 12-4 ). It is recognized that hyperalgesia can be produced by sustained inflammation, as in the case of sunburned skin. Clinical observation has shown that the sensitivity of dentin often increases when the underlying pulp becomes acutely inflamed, and the tooth may be more difficult to anesthetize. This is due in part to the upregulation of tetrodotoxin-resistant (TTX-resistant) sodium channels in inflamed neural tissue. NGF seems to play an important role in hyperalgesia. NGF regulates chronic inflammatory hyperalgesia by controlling gene expression in sensory neurons, including genes involved in inflammatory hyperalgesia in the dental pulp. Although a precise explanation for hyperalgesia is lacking, apparently localized elevations in tissue pressure and inflammatory mediators that accompany acute inflammation play an important role. Clinically, we know that when the pulp chamber of a painful tooth with an abscessed pulp is opened, drainage of exudate soon produces a reduction in the level of pain. This suggests that mechanical stimuli may contribute substantially to pain during inflammatory hyperalgesia.
Signs of Hyperalgesia | Related Diagnostic Tests or Symptoms |
---|---|
Spontaneous pain | Spontaneous pain |
Reduced pain threshold | Percussion test, palpation test, throbbing pain |
Increased response to painful stimuli | Increased response to pulp test (electric or thermal test) |
From a clinical point of view, thermal allodynia is the term that best describes a patient whose chief complaint is “I have pain when I drink cold beverages.” Mechanical allodynia is involved when the chief complaint is “It now hurts when I bite on this tooth.” These previously non-noxious stimuli now cause the perception of pain. Hyperalgesia is manifested in endodontic pain patients when noxious stimuli (e.g., refrigerant sprays or carbon dioxide snow used in the cold test) produce much more pain than they would in teeth with normal pulp tissues. Spontaneous pain involves episodes of pain that seem to be unprovoked. All these changes can be partly explained by sensitization of peripheral nerve endings in the pulp and periradicular tissues.
Many silent nerve fibers are present in the normal pulp and are termed silent because they are not excited by ordinary external stimuli. Once they are sensitized through pulpal inflammation, they begin to respond to hydrodynamic stimuli. This phenomenon may provide an additional mechanism for dentin hypersensitivity. The molecular mechanisms of this activation are not known in detail but involve upregulation of numerous genes and their products.
Inflammatory Mediators
Among the best characterized of the inflammatory mediators are the prostaglandins (PGs), which are derived from arachidonic acid via the action of the cyclooxygenase (COX) enzyme systems. The human COX enzyme is known to exist in at least two forms, COX-1 and COX-2. COX-1 is constitutively expressed and produces PGs that are involved in basic housekeeping functions such as cytoprotection in the stomach, regulation of blood flow in the kidneys, and the formation of thromboxane A 2 . The formation of thromboxane A 2 can ultimately lead to platelet aggregation; therefore, inhibition of thromboxane A 2 should decrease platelet aggregation. COX-2 is inducible, synthesized in inflamed tissues (including dental pulp), and is important in the production of the proinflammatory PGs as well as the vasodilating prostacyclin (PGI 2 ). Although they do not produce pain if applied alone, PGs are known to sensitize peripheral nociceptors, which increases the algogenic (pain-producing) properties of serotonin and bradykinin. The exact mechanism by which PGs increase neuronal excitability is not clear, but there is a growing body of evidence to suggest that they activate the PG E receptor subtypes EP 2 and EP3 in the trigeminal system and exert their effects by regulating the activity of certain ion channels, including voltage-gated sodium channels (for a review, see England ). For example, application of prostaglandin E 2 (PGE 2 ) to isolated dorsal root ganglion neuronal somata more than doubles the responsiveness of certain sodium channels found predominantly on nociceptors—channels thought to be relatively resistant to lidocaine. When administered to rats before an inflammatory insult, ibuprofen, a nonselective COX inhibitor, has been shown to block the increased expression of Na v 1.7 and Na v 1.8. Therefore, if the concentrations of PGs in inflamed pulp and periradicular tissues can be decreased with nonsteroidal antiinflammatory drugs (NSAIDs) or corticosteroids, postoperative pain may be relieved; in addition, more profound local anesthesia may be achieved in patients with hyperalgesia of pulpal origin. It is interesting to note that sensory neurons themselves are a source of PGs; during inflammation, the levels of PGE 2 appear to increase in dorsal root ganglia and spinal cord, suggesting that NSAIDs also have a central site of action (discussed in Chapter 4 ).
Bradykinin (BK) is a proinflammatory mediator derived from circulating plasma proteins and also causes direct activation of nociceptive neurons, resulting in pain. Increased levels of BK have been demonstrated in the inflamed dental pulp, and the presence of growth factors associated with inflammation (e.g., nerve growth factor) have been reported to cause an increase in the expression of mRNA encoding B1 and B2 receptors in primary cultures of rat trigeminal ganglia, as well as other receptors such as TRPV1 and TRPA1. The transient receptor potential subtype V1 (TRPV1) is the “capsaicin receptor”; it plays a key role in mediating inflammatory pain. TRPA1 is expressed on capsaicin-sensitive neurons and interacts with TRPV1. Bradykinin likely increases the excitability of nociceptive neurons through its action on TRPV1 and TRPA1 (for review, see Tominaga et al ).
Cytokines are a diverse group of regulatory proteins synthesized and secreted by a variety of cell types, such as leukocytes, neurons, and glia. In particular, tumor necrosis factor α (TNF-α) and the interleukins IL-1β, IL-6, and IL-8 are thought to play a role in the neuroplastic changes that occur in nociceptors innervating inflamed tissues, leading to hyperalgesia. Application of TNF-α rapidly sensitizes TRPV1, contributing to activation of the capsaicin-sensitive class of nociceptors. All of the above are thought to exist in the inflamed pulp (for review, see Fouad ) and are thought to act at least in part by causing increased release of prostanoids.
Painful Pulpitis
From the foregoing, it is apparent that pain associated with the stimulation of the A fibers does not necessarily signify that the pulp is inflamed or that tissue injury has occurred. Clinically, pain produced by A fibers in response to the hydrodynamic mechanism has a sharp or bright quality as contrasted with the dull, boring, or throbbing pain associated with C fibers. A fibers have a relatively low threshold of excitability to external stimuli, and painful pulpitis is more likely to be associated with nociceptive C fiber activity indicative of pulpal tissue injury. The clinician should carefully examine symptomatic teeth to rule out the possibility of hypersensitive dentin, cracked or leaky fillings, or fracture lines—each of which may initiate hydrodynamic forces—before establishing a diagnosis of reversible or irreversible pulpitis (see also Chapters 1 and 21 ).
Pain associated with an inflamed or degenerating pulp may be either provoked or spontaneous. The hyperalgesic pulp may demonstrate a lowered threshold of pain by responding to stimuli that usually do not evoke pain (allodynia), or the pain may be exaggerated and persist longer than normal (hyperalgesia). On the other hand, the tooth may commence to ache spontaneously in the absence of any external stimulus. Spontaneous, unprovoked pain generally indicates a pulp that is seriously damaged and generally will not respond to noninvasive therapy.
Plasticity of Intradental Nerve Fibers
It has become apparent that the innervation of the tooth is a dynamic process in which the number, size, and cytochemistry of nerve fibers can change because of aging, tooth injury, and dental caries. For example, in rats, nerve fibers sprout into inflamed tissue surrounding sites of pulpal injury, and the content of CGRP and SP increases in these sprouting fibers. When inflammation subsides, the number of sprouts decreases. Fig. 12-30 compares the normal distribution of CGRP-immunoreactive sensory fibers in an adult rat molar with those beneath a shallow cavity preparation. The innervational pattern in normal and inflamed teeth is governed by neuronal growth factors. Neurotrophic and target-derived factors regulate neuronal structure, survival, and function and are important for the maintenance of neuronal phenotype characteristics. During development, all dental fibers appear to require nerve growth factor (NGF) and express its receptor, TrkA, at some stages, whereas in adult teeth the large trigeminal neurons are potentially dependent only on dental pulp–derived, glial cell line–derived neurotrophic factor (GDNF); the smaller trigeminal neurons remain dependent on NGF. This suggests that GDNF may function as a neurotrophic factor for the subset of larger neurons supporting the tooth, which apparently mediate mechanosensitive stimuli, whereas NGF is suggested to support neurons responsible for nociception. NGF is the most extensively investigated among the trophic factors. Binding of target-derived NGF is dependent on specific TrkA receptors located on the axonal surface, with subsequent internalization and transport to the cell body, where the effects are mediated.
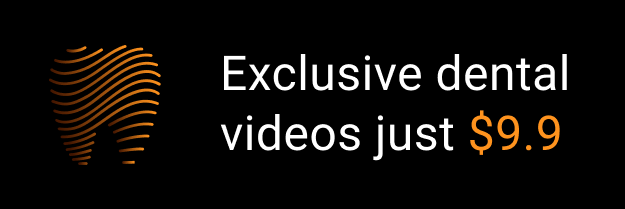