Successful periodontal regeneration requires the hierarchical reorganization of multiple tissues including periodontal ligament, cementum, alveolar bone, and gingiva. The limitation of conventional regenerative therapies has been attracting research interest in tissue engineering-based periodontal therapies where progenitor cells, scaffolds, and bioactive molecules are delivered. Scaffolds offer not only structural support but also provide geometrical clue to guide cell fate. Additionally, functionalization improves bioactive properties to the scaffold. Various scaffold designs have been proposed for periodontal regeneration. These include the fabrication of biomimetic periodontal extracellular matrix, multiphasic scaffolds with tissue-specific layers, and personalized 3D printed scaffolds. This review summarizes the basic concept as well as the recent advancement of scaffold designing and fabrication for periodontal regeneration and provides an insight of future clinical translation.
Key points
- •
Periodontal regeneration requires the hierarchical reorganization of soft and hard tissues, namely, periodontal ligament, cementum, alveolar bone, and gingiva.
- •
Three-dimensional microporous scaffolds offer structural support and spatiotemporal guidance for cell growth and differentiation.
- •
Biomimetic periodontal extracellular matrix scaffold may be produced by combining periodontal ligament cells and microporous scaffolds with the prospect of off-the-shelf products.
- •
Selection of scaffold architecture, functionalization techniques, and cell types determines the functionality of scaffolds.
- •
Three-dimensional printing technology allows for designing personalized scaffolds for periodontal regeneration.
Introduction
Advanced periodontitis results in the damage and loss of hard and soft tissues, which impairs oral function, aesthetics, and the patient’s overall quality of life. Although conventional therapies such as scaling and root plaining and flap surgery effectively interrupt disease progression, it often necessitates regenerative interventions to regain the original architecture and function of periodontal tissues because of limitation in spontaneous regeneration. , This requires newly formed cementum and alveolar bone bridged by functional periodontal ligament. Conventional regenerative approaches aim at promoting the growth and differentiation of tissue-resident progenitor cells into fibroblasts, cementoblasts, and osteoblasts, while preventing the downgrowth of epithelial tissues into the periodontal defect. This approach, termed guided tissue regeneration, is represented by the application of barrier membranes with or without bioactive molecules such as enamel matrix derivative and recombinant growth factors. Additionally, autogenous bone or bone substitutes of allogeneic, xenogeneic, or alloplastic origin, may be applied as scaffolds for cell growth and migration. These interventions have been shown to be effective clinically. However, a large heterogeneity among studies affirms the unpredictability of the treatments, and none of the existing treatment options have achieved complete periodontal regeneration. ,
A conventional regenerative strategy, namely, bone grafting, mainly uses autogenous bone and various bone substitutes. Autogenous bone is considered as the golden standard because it has osteoconductive, osteoinductive, and osteogenic properties attributed to the components: autologous cells (eg, osteoblasts and their progenitor cells), extracellular matrix (ECM) components (eg, collagen, hydroxyapatite), and bioactive molecules (eg, bone morphogenetic protein-2 [BMP-2]). However, owing to limitations in the amount of harvestable bone and the necessity of surgical intervention to donor sites, the use of allografts and xenografts, which are obtained from a donor of the same or different species, respectively, have been preferred as alternatives. Nevertheless, they carry the risk of unforeseen infection, disease transmission, and/or immune rejection. In contrast, alloplastic or synthetic bone substitutes, which are mostly made from hydroxyapatite, for example, tricalcium phosphate, calcium sulfate, biphasic calcium phosphate, possess osteoconductivity, but are not of biological origin and, therefore, do not carry the risk of disease transmission. Bone substitute materials are delivered to osseous defects, including periodontal defects as scaffolds, and their osteoconductivity is hypothesized to stimulate endogenous progenitors to grow and differentiate into mature osteoblasts. However, a systematic review has revealed that the outcome of periodontal therapy solely with bone grafting, that is, without accompanying barrier membranes, is predominantly ascribed to bone regeneration with an attachment of long junctional epithelium, but with a lack of newly formed cementum and periodontal ligament. Moreover, the effect of such combinational therapies seems to be limited in horizontal and 2-walled intrabony defects, and inadequate in 3-walled intrabony and advanced furcation defects.
Although conventional approaches to periodontal regeneration predominantly rely on the regenerative capacity of endogenous cells, the comparatively newer tissue engineering approach aims to combine exogeneous progenitor cells, biomaterial scaffolds, and bioactive molecules (signals) to address the complex architecture and function of the periodontal tissues. , In nature, ECM possesses optimal structural patterns and bioactivity, which regulate the growth and fate of the residing cells spatiotemporally. Meanwhile, the concept of biomimetics was brought into the fabrication of tissue engineered constructs for periodontal regeneration. Despite a large variance among studies, most of the designing concepts converge in mimicking the hierarchical organization of the native periodontal tissues, particularly the ECM, structurally and functionally in an ex vivo setting. Scaffolds, therefore, serve as the core of tissue engineered construct because they offer 3-dimensional (3D) structural support and spatial guidance for cells. Moreover, their functionality may be further enhanced by incorporating bioactive molecules, for example, growth factors. A wide variety of conventional and state-of-art scaffold fabrication methodologies such as decellularization, salt leaching, electrospinning, and 3D printing have been tested to fabricate biomimetic scaffolds to challenge the complex nature of periodontal tissues. The aim of this article is to review the concepts of scaffold designing and fabrication, and to summarize the recent advancements in tissue engineering-based applications of biomimetic scaffolds for periodontal regeneration.
Scaffold designing and fabrication concept for periodontal regeneration
Scaffolds act as the core of tissue-engineered constructs because they offer spatiotemporal guidance for cells by providing architectural and biochemical clues. Scaffold designing requires the selection of material, fabrication techniques, and functionalization methods ( Fig. 1 ). This section summarizes scaffold designing and fabrication concept for periodontal applications.

Scaffold Architectures and Fabrication Techniques
Scaffolds provide the structural support and the guidance for exogenous and/or endogenous cells. Generally, 3D scaffolds with high porosity and interconnectivity are preferable to achieve structural and functional restoration, because the architecture offers a suitable microenvironment for cell-to-cell interaction and scaffold-to-tissue integration at the implanted site. , In the early phase of implantation, the porous structures facilitate blood infiltration to the scaffolds and stabilize the blood clots, which is considered as a key initiator of tissue repair and regeneration through enriched vascularization. , Particularly, macropores ranging from 100 to 700 μm enhance vascularization at the implanted sites, whereas micropores of less than 100 μm may suppress cell growth owing to local ischemia. , High porosity also supports the diffusion of nutrients and gases as well as waste removal, which improves cellular metabolism and growth. Various fabrication techniques have been used to design highly porous scaffolds. ,
In nature, the ECM has an amorphous porous structure, acting as a scaffold. It regulates the recruitment, growth, and differentiation of resident cells via bioactive molecules, spatial patterning, and mechanical stimuli. As an exogeneous complete form of ECM, decellularized ECM are widely applied to reproduce a 3D microenvironment at the implanted sites for tissue repair and regeneration. Decellularized ECM products from various origins, including human, porcine, or bovine dermis, and human amniotic membrane (hAM) are commercially available and used in clinical practice. Recently, donor sites have been extended to the periodontal ligament itself, and attempts have been made to produce biomimetic periodontal scaffolds using decellularized ECM in combination with periodontal progenitor cells. To reproduce the structural pattern of ECM artificially, various techniques have been translated into regenerative medicine. Salt leaching, gas forming, phase separation, and freeze drying are representative conventional methods to produce highly porous amorphous scaffolds (see the previous review on fabrication methods). Salt leaching and gas forming techniques use salt and gas as porogen additives, whereas phase separation and freeze drying techniques use volatilization and sublimation of solvent and/or water in the polymer solution. Electrospinning is one of the representative engineering techniques used to produce fine fibrous scaffolds. It generates nonwoven nanoscaled-to-microscaled fibers, which reportedly mimic the native collagen fibrous network. The electrospinning process requires a solvent–polymer mixture in a syringe pump, a collector, and a high voltage supply. When the high gradient of electric potential difference is applied between the metal syringe tip and the collector, electric charge accumulates on the polymer solution at the needle tip, and the polymer solution is ejected as a liquid jet toward the collector. When the jet reaches the collector, the polymer gets solidified because of evaporation of the solvent. These techniques allow for the fabrication of highly porous scaffolds, and resulting structures incorporate pores with various shapes and sizes, as if to recapitulate the structural pattern of the native ECM. However, the controllability of internal architecture, that is, pore size, pore distribution, and pore orientation, is relatively low in comparison with rapid prototyping methods represented by 3D printing. Furthermore, the resulting structures are considered as monophasic; it is characterized by the consistency in overall physical and chemical properties within the structures ( Fig. 2A ).

Provided that periodontal regeneration requires the hierarchical orientation of multiple tissues, a multiphasic design (ie, biphasic or triphasic) is considered to direct progenitors to specific cell types more rigorously ( Fig. 2 B). This structure can be designed by layering components with different characteristics such as material composition, architectures, and functionalization. , Depending on designing concepts, countless combinations are possible. Although there is no perfect combination, a number of proof-of-concept studies have developed prototype designs that potentially favor the regeneration of the hierarchical structures. In particular, 3D printing technology has recently emerged as a promising strategy to produce multilayered constructs for tissue engineering, because it overcomes the limitation of conventional fabrication techniques. Namely, difficulties in precise structural control and reproducibility are avoided. Moreover, recent advances in bioprinting have empowered the possibility of producing functional artificial organs in vitro. With the help of 3D computer-aided design (CAD) modeling software, constructs can be built up in a layer-by-layer fashion in accordance with the blueprints. Currently, 3D printers for biomedical applications can achieve a minimum of 10 μm resolution with high accuracy. The major advantage of 3D printing for scaffold fabrication is attributable to its designing flexibility. Controlling porosity, pore size, interconnectivity, and strand alignment pattern creates structural gradient within the construct, which may guide tissue orientation. The 3D printed scaffolds could be produced in a monophasic or multiphasic form depending on its design. Further advantage of 3D printing derives from its compatibility with diagnostic imaging equipment such as a cone beam computed tomography (CT) scan and intraoral 3D scanner. The geometry of periodontal defects is scanned and transferred into CAD modeling software to produce custom-designed 3D scaffolds adapting to the defect. This personalized medicine approach is expected to increase the predictability of periodontal therapy for advanced tissue defects.
Polymeric Scaffold Materials and Functionalization
Material development and scaffold designing have been the major interest in biomaterial research for regenerative medicine. Although natural ECM serves as the ideal scaffold in nature, particular attention has been paid to the generation of biomimetic scaffolds using polymeric biomaterials. Polymeric biomaterials possess biodegradability and biocompatibility, which allow the materials to be used for a wide range of medical application as implants for soft and hard tissue regeneration. Polymeric biomaterials are categorized based on their origin: natural and synthetic polymers.
Natural polymers represented by proteins (eg, collagen, silk) and polysaccharides (eg, cellulose, alginate, chitosan) are often referred as the first biodegradable biomaterials applied in clinical settings. They possess inherent bioactive properties that actively interact with cellular components. For example, integrin-binding ligands are presented on protein-based polymers, which regulate cell adhesion, migration, proliferation, and differentiation. However, natural polymers generally lack mechanical stability, and their mechanical/biological properties may significantly vary depending on extraction procedures. Furthermore, their high susceptibility to enzymatic degradation may result in disharmonized scaffold resorption and tissue remodeling. Therefore, reinforcement with resilient materials such as fibers or hydroxyapatites is often considered.
In contrast, synthetic polymers such as polylactic acid, polycaprolactone (PCL), and poly( dl -lactide- co -glycolide) present superior mechanical properties and formability for clinical use in a variety of applications in addition to decent biocompatibility and biodegradability. By altering molecular weight and chemical composition, favorable biodegradability and mechanical properties are delivered to the scaffold. However, unlike natural polymers synthetic polymers are biologically inert, and their hydrophobic nature may hinder blood infiltration, which potentially prevents the scaffold from integrating to the implanted site. To supplement the bioinertness of the synthetic polymers, functionalization using techniques such as plasma surface activation and the coating/additive of bioactive molecules are preferably performed. , These include ECM proteins (eg, collagen, fibronectin, gelatin), growth factors (eg, BMP-2, BMP-7, fibroblast growth factor-2, and platelet-derived growth factor BB), , specialized proresolving mediators (eg, resolving D1), and various types of antibiotics and anti-inflammatory drugs. Generally, functionalization to the synthetic polymer does not alter the bulk property of the materials but increases interaction between material and tissues. With this background in scaffold design and fabrication, the subsequent sections discuss the applications of various scaffold-based tissue engineering strategies in experimental settings for periodontal regeneration.
Monophasic scaffold approaches for periodontal regeneration
Decellularized Extracellular Matrix as an Exogeneous Natural Matrix
ECM is a natural form of complete scaffold, providing a suitable biochemical and biomechanical microenvironment for the residing cells. In a current clinical practice, an autologous connective tissue graft (CTG) is a frequent procedure to augment soft tissue. In addition to soft tissue regain, a histologic evaluation has revealed that CTG leads to the regeneration of cementum on the dentin surface, which may be bound to newly formed periodontal ligament, indicating connective tissue exhibits cementoconductivity. However, the procedure is accompanied by a number of complications not only at the recipient site, but at the donor site such as pain, infection, bleeding, and necrosis.
To overcome the limitation of the autologous soft tissue graft, decellularized ECM from allogenic or xenogeneic origin have been an alternative ( Fig. 3 ). Acellular dermal matrix (ADM) from human skin is the most common decellularized ECM scaffold in periodontal treatment. Although most of the clinical application in dentistry is limited to periodontal plastic surgery, its cementoconductivity and osteoconductivity supported by periodontal ligament regeneration has been suggested by in vivo models. A study using mini pigs with surgically created fenestration defects showed that clinical attachment gain by ADM was comparable with by CTG after 3 months of healing period, but ADM implantation led to greater new cementum regeneration with the narrower length of epithelial and connective tissue attachment. The regenerative capacity of ADM may be further enhanced by combining bone substitute, as shown previously in beagle dogs that ADM in combination with beta-tricalcium phosphate induced the greater periodontal regeneration with thick cementum layers and alveolar bone formation that were bridged by periodontal ligament than ADM alone and coronally repositioned flap surgery. Similarly, xenogeneic decellularized matrix possess comparable effects on the regeneration of periodontal tissue, although soft tissue response may differ. , , There is a lack of evidence in the use of ADM to intrabony or furcation defects, but it supports the adhesion, robust proliferation of human periodontal ligament cells (PDLC) and possesses optimal biocompatibility and biodegradability for periodontal regeneration, suggesting its potency as a scaffold material.

The hAM obtained from maternal donors undergoing caesarian section is another source of allogenic ECM, mainly for soft tissue repair and regeneration. There is an absence of blood vessels and lymphatic tissue in hAM, and it has high durability and superior mechanical property attributed to the tight network of collagen and elastin fibers. Human AM has been proven to contains rich growth factors such as epithelial growth factor, basic fibroblast growth factor, transforming growth factor-α and -β, vascular endothelial growth factor, and hepatocyte growth factor, all of which are positively corelated to periodontal regeneration through anti-inflammatory effects, immunomodulatory effects, antibacterial effects, and promotion of endogenous progenitor growth. On hAM, PDLC are able to maintain their phenotype as in vivo with robust expression of ki-67, vimentin, desmoplakin, and ZO-1, but not keratins 4 and 13, suggesting its compatibility for periodontal regeneration. It was shown that the use of hAM as a barrier membrane in combination with hydroxyapatite granules had advantageous effects on the suppression of the local inflammation at the recipient site, resulting in greater clinical attachment gain with increased bone generation than the bone substitutes only. , Although the efficacy of hAM alone to induce periodontal regeneration remains elusive, it was proven to be a promising scaffold for cell-based periodontal therapy. , An in vivo study in immunodeficient mice showed that hAM loaded with periodontal ligament stem cells (PDLSC) induced bone regeneration in surgically created class II furcation defects. The histologic analysis confirmed new cementum formation, with single-layered cementblast-like cells on the surface, in which Sharpey’s fibers were inserted. Similarly, the transplantation of adipose-derived mesenchymal stem cells on hAM regenerated 2-wall osseous defects in a rat model. These results confirmed that hAM supported cementogenesis, osteogenesis, and fibrogenesis in experimental periodontal defects.
The ECM could also be obtained from dental tissues. Indeed, a detailed protocol to harvest and decellularize ECM from dental tissues without deteriorating intermingled collagen fiber networks have been recently reported, and it was successfully applied to periodontal tissues. , Naturally, the ECM of periodontal origin could be considered to possess the ideal microenvironment (eg, topography, protein composition) for periodontal regeneration. An in vitro study investigating the fatal determination of PDLSC on decellularized periodontal ECM from tooth slice has indicated its unique usability as a scaffold. In the study, decellularized periodontal ECM was repopulated by PDLCS. Strikingly, PDLSC that were found near the decellularized cementum layer selectively expressed cementoblast markers, cementum protein-23 and osteocalcin, while keeping fibrous network within the decellularized area of periodontal ligament. This finding has confirmed that decellularized periodontal tissues maintains spatial information, which may guide the fate of PDLSC. Although no study has tested the regenerative capacity of decellularized periodontal EMC in periodontal defects, a tooth replantation model in beagle dogs has suggested that it potentially regenerates periodontal tissues structurally and functionally. In the study, mandibular premolars were extracted and processed to decellularize the residual periodontal tissues on the root surface. The teeth were then replanted in the surgically expanded extraction socket. Interestingly, there was no significant difference between the freshly extracted teeth and the decellularized teeth in root resorption, recovered periodontal ligament area and new cementum formation. The study also showed rich revascularization in the decellularized matrix, suggesting that decellularized periodontal ECM was sufficient to retrieve its hierarchical structure and function by recruiting endogenous progenitors.
Nevertheless, the clinical translation of decellularized ECM originated from periodontal tissues seems challenging although periodontal ligament can be obtained from deciduous teeth, wisdom teeth, and extracted teeth for orthodontics treatment and then cryopreserved. The technique requires the provision of infrastructure, namely, “tooth banks,” and improved cost efficiency before being manufactured as off-the-shelf products for example, ADM and hAM.
Bioengineered Periodontal Extracellular Matrix as a Biomimetic Approach
Contrary to natural ECM-based approaches, bioengineering techniques may be used to produce biomimetic periodontal scaffolds in combination with progenitor cells and/or bioactive molecules. A nanotopographical pattern of scaffolds, such as pores, grooves, and ridges, regulates cell growth, mobility, and fate. Various techniques have been used to produce biomimetic ECM which has close resemblance to natural fibrous ECM. In particular, electrospinning has caught appreciable attention because of the unique features of the end products. Electrospun scaffolds consist of nonwoven nano-to-micro filaments with favorable porosity and interconnectivity for cell growth, which resemble to the structural pattern of natural ECM. It is compatible with various natural and synthetic polymers, and further functionalization may be combined by adding bioactive molecules in the melts.
Electrospun constructs have been used as a substrate to produce biomimetic periodontal ECM in combination with progenitor cells ( Fig. 2 B). Simply, PDLC seeded on an electrospun substrate were able to produce ECM by secreting collagen I, fibronectin, and rich growth factors, which are found in native periodontal tissues, such as basic fibroblast growth factor, vascular endothelial growth factor, and hepatocyte growth factor. , Importantly, the secreted proteins were preserved on the substrate after the decellularization process, indicating that the engineered construct mimicked the architecture and function of the native periodontal ECM. , Furthermore, the electrospun substrate provided a structural reinforcement during production process, which prevented the construct from being deformed and damaged during production process. This allowed for further preclinical assessment of the biomimetic periodontal ECM in surgically created periodontal fenestration defects in rat, showing that it significantly promoted the regeneration of periodontal ligament, cementum, and alveolar bone in comparison to electrospun PCL scaffolds alone. It has been shown that decellularized bioengineered ECM did not show immunogenicity, and it could be repopulated by allogenic and xenogeneic cells , , , Therefore, the concept of biomimetic periodontal ECM has a potential to be clinically transferred as off-the-shelf products.
The idea of biomimetic periodontal ECM may be further enhanced by controlling nanofiber orientation. On parallelly aligned PCL electrospun nanofibers, PDLC upregulated the expression of periostin, which regulates homeostasis of periodontal tissues in response to occlusal load. , Further in vivo observation in a periodontal fenestration defect model in rat showed that aligned PCL electrospun nanofibers loaded by PDLC noticeably regenerated periodontal ligament, which was perpendicularly oriented to the root surface, whereas randomly aligned nanofibers resulted in irregular ligament orientation. This finding suggests that fiber orientation governs the architecture and function at the regenerated sites.
The functionalization of electrospun constructs may also expand the feasibility of hierarchical periodontal regeneration. For example, adding collagen type 1 and nano-hydroxyapatites in PCL solution before extrusion allowed the end product to be osteoinductive, promoting the expression of alkaline phosphatase and osteocalcin expression by PDLCs. Provided that periodontitis is an inflammatory disease caused by bacterial infection, functionalization with nonsteroidal anti-inflammatory drugs or antibiotics in anticipation of sustained drug release seems valid. The immobilization of nonsteroidal anti-inflammatory drugs such as meloxicam and ibuprofen in electrospun fibers allowed the construct to possess a long-term anti-inflammatory effect. Interestingly, PCL electrospun scaffolds functionalized with ibuprofen selectively suppressed the proliferation of gingival cells subjected to Porphyromonas gingivalis lipopolysaccharide. In an experimentally induced periodontitis model, PCL electrospun scaffolds functionalized with ibuprofen significantly decreased local inflammation and further progression but improved the clinical attachment level in comparison with the nonfunctionalized counterpart. Functionalization with antibiotics such as doxycycline hydrochloride, metronidazole, and tetracycline hydrochloride has been also suggested to be efficacious against the progression of periodontitis and to provide better sustainability after implantation. These functionalization techniques do not alter the bulk properties of the polymeric scaffolds, but may offer additional benefits to periodontal regenerative therapy.
Multiphasic scaffold approach for targeting tissue-specific regeneration
Periodontal regeneration requires the spatiotemporal reorganization of newly formed periodontal ligament, cementum, and alveolar bone. These components are suggestive of porous medium with approximately 70% to 90% porosity, but each component has unique cellular components, matrix pattern, and functionality. Therefore, multiphasic scaffolds are designed to consist of multiple components layer by layer, each of which specifically targets their corresponding tissue. There are countless designing concepts to achieve compartmentalized periodontal regeneration; it can be the combination of differential materials, architectural patterns, functionalization, and cell types.
Biphasic scaffolds are often designed to combine bone compartment and periodontal compartment. Vaquette and colleagues (2012) developed double-layered PCL scaffolds which consisted of a bone compartment produced by 3D printing and an electrospun periodontal compartment. In this study, osteoblasts in suspension and PDLC in sheet were loaded on their corresponding components, and then the construct was placed on a dentin block as the periodontal compartment was in contact with the dentin surface before subcutaneous transplantation in an immunodeficiency rat model. The histologic evaluation noted that a cementum-like tissue was formed on the dentin surface in which fibrous attachment supported, whereas the expression of alkaline phosphatase was promoted on the bone compartment side. The following study further optimized the scaffold design by functionalizing the bone compartment with calcium phosphates, showing improved bone formation. The other common biphasic approach is to combine a barrier membrane and porous scaffold as one unit. Despite differences in designing concept among studies, it would be concluded the concept may improve the tissue regeneration in comparison with barrier membrane or scaffold alone. However, further comparative studies between the biphasic scaffold approach and the conventional combination of barrier membrane and graft material separately are needed to verify its additional therapeutic benefit.
Triphasic scaffolds are mostly designed to individually target each of 3 components in periodontal tissues to provide more specific spaciotemporal guidance. Despite complexity in fabrication methods, several studies have successfully produced triphasic scaffolds for periodontal application. For example, 3D printing technology facilitates producing triphasic scaffolds by changing strand alignment patterns. Lee and colleagues (2014) verified the triphasic concept by the orthodox tissue engineering approach, namely, by combining scaffolds, bioactive molecules, and progenitor cells. In this study, triphasic 3D printed scaffolds of nanohydroxyapatite-containing PCL were designed by changing porous patterns. Three phases were designed with 100 μm, 600 μm, and 300 μm microchannels to approach cementum/dentin interface, periodontal ligament, and alveolar bone, respectively. Subsequently, layers for the cementum–dentin interface, periodontal ligament, and alveolar bone were functionalized with human amelogenin, connective tissue growth factor, and BMP-2, respectively, before the scaffold was loaded by dental pulp stem cells and transplanted subcutaneously in immunodeficient mice. Notably, phase-specific tissue formation was observed: dense and polarized mineral formation with the upregulation of dentin sialophosphoprotein and cement matrix protein 1 in the cementum/dentin phase, aligned fibrous matrix formation with the upregulation of collagen type 1 in the periodontal ligament phase, and scattered mineral formation with the upregulation of bone sialoprotein in the bone phase. This finding suggests that multiphasic structures effectively guide tissue-specific regeneration by providing optimal microstructure and spatiotemporal delivery of bioactive molecules. Another example is the combination of differential cell types and mechanical properties. Varoni and colleagues (2017) produced chitosan-based porous scaffolds that consisted of bone and periodontal layers produced by the freeze-drying method and a dense mesh gingival layers by electrochemical deposition. Differential stiffness was given to each of the layers by controlling molecular weight of cross-linking reagent: a stiff layer aiming for bone regeneration and a soft layer for the interaction with the gingiva and the periodontal ligament. Osteoblast, PDLC, and gingival fibroblast were then seeded to their corresponding layers. In an in vivo ectopic periodontal model, as expected, robust expression of tissue specific markers was found: periostin and collagen type 1 in the periodontal ligament layer, osteopontin and bone sialoprotein in the bone layer, and cement matrix protein-1 adjacent to the dentin surface. Also, putative cementum and bone were newly formed in the bone and periodontal layers.
It is also relevant to mention other studies that have introduced novel fabrication techniques for triphasic scaffolds that may potentially guide hierarchical regeneration, albeit without testing their in vivo efficacy. , , Nevertheless, scaffold fabrication is complicated with each additional phase, particularly with regard to small scaffolds for periodontal applications, and a lack of in vivo evidence for the efficacy of such complex scaffolds precludes a conclusion on their current clinical relevance.
Custom-designed 3-dimensional scaffold for a personalized periodontal approach
A personalized medicine approach underlies the concept of pathologic variation among patients. Optimal periodontal regeneration requires spatial guidance to progenitor cells with rich vascularization while preventing epithelial downgrowth. Therefore, 3D scaffolds with defect-specific geometry may enhance periodontal regeneration. This goal could be achieved by applying a medical imaging system such as a high-resolution cone beam CT scan in scaffold designing. The prototype workflow of custom-designed 3D scaffolds for periodontal regeneration was introduced by Park and colleagues 49,63 (2010, 2012). Surgically created periodontal fenestration defects were scanned by a micro-CT scan, and the scanned files were then transferred into CAD software as 3D image data in .stl format, where the scaffold geometry was designed to adapt to the defect. In the scaffold, microchannel architectures were included in the scaffold to provide an orientational guide to periodontal ligament fibers. Subsequently, a wax mold was created by a wax printer, and PCL was casted in the mold. , After the sterilization process, PDLC were loaded on the custom-designed scaffold and transplanted to the defect site. After 4 weeks of healing, the custom-designed scaffold resulted in a significant increase in bone mass and mineral density, and the alignment of regenerated periodontal ligament was oriented more regularly in comparison with amorphous scaffolds with random pores produced by the freeze-drying method. Strikingly, the expression of periostin, which is the regulator of collagen fibrogenesis found in functional periodontal ligament, was evident in the treated site by the custom-designed scaffold, but not by the amorphous scaffold. The development of high-resolution 3D printing technology has facilitated the fabrication of on-demand scaffolds ( Fig. 4 ). The clinical implication of the approach was reported by Rasperini and colleagues (2015) in which a fenestration defect in the mandibular canine was treated by 3D printed custom-designed scaffold. The scaffold was designed based on CT data and printed by selective laser sintering. During periodontal surgery, the scaffold was immersed in recombinant human platelet-derived growth factor BB and then transplanted in the defect site. After 12 months of the treatment, clinical attachment gain and radiological bone regeneration was observed without complication. In this case, however, the scaffold was exposed at 13 months, and then the entire scaffold was removed owing to infection. After histologic assessment and gel permeation chromatography showed that a great amount of the scaffold was still found with approximately 76% of PCL molecular weight remained. Also, the new bone formation was limited. This suggests that custom-designed scaffold may guide the tissue regeneration, but the prognosis largely depends on concordant material degradation and biological interaction between materials and tissues. Further optimization of internal microstructure, polymer selection, and polymer functionalization may contribute to an improved outcome.
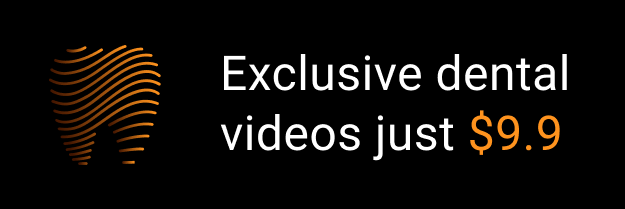