Resin-Based Composites
History
There have been remarkable developments in filler, bonding, and curing technologies in esthetic restorative materials over the past 55 years, as shown in Figure 13-1. During the first half of the twentieth century, silicates were the tooth-colored material of choice for cavity restoration. Silicates release fluoride and are excellent for preventing caries, but they are currently used almost exclusively for deciduous teeth because they become severely eroded within a few years (see Chapter 14). Acrylic resins, similar to the materials used to make dentures and custom impression trays (polymethylmethacrylate [PMMA], see Chapter 20), soon replaced silicates because of their tooth-like appearance, insolubility in oral fluids, ease of manipulation, and low cost. Unfortunately, these acrylic resins had relatively poor wear resistance and tended to shrink severely during curing, which caused them to pull away from the cavity walls, thereby producing crevices or gaps that facilitiate leakage within these gap. Excessive thermal expansion and contraction caused further stresses to develop at the cavity margins when hot or cold beverages and foods were consumed.
In 1962, Bowen developed a new type of composite material that largely overcame these problems. Bowen’s main innovations were bisphenol-A glycidyl dimethacrylate (bis-GMA), a monomer that forms a cross-linked matrix that is highly durable (see Chapter 6), and a surface treatment utilizing an organic silane compound called a coupling agent to bond the filler particles to the resin matrix. Current tooth–colored restorative materials continue to use this technology, but many further innovations have been introduced since 1962.
Many of these advances have occurred through developments in the filler component. The filler has evolved to ever smaller sizes, mainly in order to improve appearance and polishability. Barium and other specialty glass and inorganic mineral fillers have been developed to impart radiopacity, enhance manipulation and handling, offset curing shrinkage, and improve mechanical properties. In the 1970s a category now known as traditional composites (also known as conventional or macrofilled composites) was developed; this contained very large particles of ground amorphous silica and quartz (Figure 13-2), which imparted significant improvements in mechanical properties, water sorption, polymerization shrinkage, and thermal expansion compared with unfilled acrylic. However, these composites suffered from roughening of the surface as a result of the selective abrasion of the softer resin matrix surrounding the harder filler particles. To improve surface smoothness and retain or improve the physical and mechanical properties of traditional composites, small-particle–filled composites were developed, using inorganic fillers ground to a size range of about 0.5 to 3 µm but with a broad size distribution (Figure 13-3), allowing a higher filler loading (80% to 90% by weight and 65% to 77% by volume). This resulted not only in smoother surfaces but also greater wear resistance and some decrease in curing shrinkage. Today, further advances in the filler component have resulted in microfilled composites and nanocomposites, hybrid composites, and packable and flowable composites, just to name a few.
The history and achievements in dental composites are outlined in Figure 13-1 and are further discussed in detail in this chapter.
Classification
A useful classification system for composites is one based on filler particle size and size distribution, as shown in Table 13-1. Subgroups and overlaps exist, particularly for the hybrid category, which combines filler from either the small or the traditional category with micro- and nanofillers. Any resin with elongated fillers (fibers, “whiskers,” filaments), with spheroidal particle fillers or fillers from two or more size ranges can in principle be considered a hybrid. Thus a single classification of hybrid composite is not very meaningful. Many modern dental composites have particle sizes less than 0.5 to 1.0 µm in combination with 10% or more by weight of micro- or nanofiller to adjust the paste to a desired viscosity/rheology so as to provide clinically useful manipulation and handling characteristics. Filler technology is discussed in detail below.
TABLE 13-1
Classification of Resin-Based Composites and Indications for Use
Class of Composite | Particle Size | Clinical Use |
Traditional (large particle) | 1- to 50-µm glass or silica | High-stress areas |
Hybrid (large particle) | (1) 1- to 20-µm glass | High-stress areas requiring improved |
(2) 40-nm silica | polishability (Classes I, II, III, IV) | |
Hybrid (midfilled) | (1) 0.1- to 10-µm glass | High-stress areas requiring improved |
(2) 40-nm silica | polishability (Classes III, IV) | |
Hybrid (minifilled/SPF*) | (1) 0.1- to 2-µm glass | Moderate-stress areas requiring optimal polishability (Classes III, IV) |
(2) 40-nm silica | ||
Nanohybrid | (1) 0.1- to 2-µm glass or resin microparticles | Moderate-stress areas requiring optimal Polishability |
(2) ≤100-nm nanoparticles | (Classes III, IV) | |
Packable hybrid | Midfilled/minifilled hybrid, but with lower filler fraction | Situations where improved condensability is needed (Classes I, II) |
Flowable hybrid | Midfilled hybrid with finer particle size distribution | Situations where improved flow is needed and/or where access is difficult (Class II) |
Homogeneous microfilled | 40-nm silica | Low-stress and subgingival areas that require a high luster and polish |
Heterogeneous microfilled | (1) 40-nm silica (2) Prepolymerized resin particles containing 40-nm silica |
Low-stress and subgingival areas where reduced shrinkage is essential |
Nanofilled composites | <100-nm silica or zirconia Homogeneous independent nanoparticles or nanoclusters |
Anterior and noncontact posterior areas |
Composition and Function
Matrix
The resin matrix in most dental composites is based on a blend of aromatic and/or aliphatic dimethacrylate monomers such as bis-GMA (see Figure 7-16) and urethane dimethacrylate (UDMA, Figure 7-18) to form highly cross-linked, strong, rigid, and durable polymer structures (see Figures 7-3 and 13-16). This matrix forms a continuous phase in which the reinforcing filler is dispersed. Because of the large molecular volume of these monomers, polymerization shrinkage can be as low as 0.9% (average of 1.5% compared with a range of 2 to 3% for most composites) when combined with inorganic particulate fillers at levels of up to 88% by weight. However, UDMA and bis-GMA are highly viscous (800,000 centipoise, similar to honey on a cold day) and are difficult to blend and manipulate. Thus, it is necessary to use varying proportions of lower-molecular-weight highly fluid monomers such as triethylene glycol dimethacrylate (TEGDMA, 5 to 30 centipoise, Figure 7-17) and other lower-molecular-weight dimethacrylates to blend with and dilute the viscous components to attain resin pastes sufficiently fluid for clinical manipulation and for incorporating enough filler to reinforce the cured resin. For example, a blend of 75% bis-GMA and 25% TEGDMA by weight has a viscosity of 4300 centipoise, whereas the viscosity of a 50% bis-GMA/50% TEGDMA blend is 200 centipoise (similar to thin syrup). Unfortunately, these smaller, diluent monomers undergo greater polymerization shrinkage, partially offsetting the advantage of using large monomers such as bis-GMA. Generally the greater the proportion of these “diluting” monomers, the greater the polymerization shrinkage and the greater the risk of eventual leakage in marginal gaps and the problems that may result.
Filler
Function of Fillers
Fillers can provide the following benefits:
• Reinforcement. Increased filler loading generally increases physical and mechanical properties that determine clinical performance and durability, such as compressive strength, tensile strength, modulus of elasticity (i.e., stiffness or rigidity), and toughness. As the volume fraction of filler approaches approximately 70%, abrasion and fracture resistance are raised to levels approaching those of tooth tissue, thereby increasing both clinical performance and durability.
• Reduction of polymerization shrinkage/contraction. Increased filler loading reduces curing shrinkage in proportion to filler volume fraction. Although shrinkage varies from one commercial composite to another, it typically ranges from slightly less than 1% up to about 4% by volume.
• Reduction in thermal expansion and contraction. Increased filler loading decreases the overall coefficient of thermal expansion of the composite because glass and ceramic fillers thermally expand and contract less than do polymers. As the overall expansion coefficient decreases with filler loading and approaches that of tooth tissue, less interfacial stress is produced because of differential volumetric changes while an individual consumes hot and cold foods and beverages.
• Control of workability/viscosity. Fluid liquid monomer + filler → a paste. The more filler, the thicker is the paste. Filler loading, filler size, and the range of particle sizes and shapes all affect markedly the consistency of a composite paste, which in turn strongly affects clinical manipulation and handling properties, such as ease of mixing and sculpting, tackiness, and the tendency to either hold a shape or to minimize slumping. Consistency and handling are more than just convenience-related properties. They determine the ease of operation, the skill and time required, and also how reliably a cavity can be restored free of errors and with the proper interproximal contact, occlusal anatomy, smoothness, and appearance.
• Decreased water sorption. Increased filler loading decreases water sorption. Absorbed water softens the resin and makes it more prone to abrasive wear and staining.
• Imparting radiopacity. Resins are inherently radiolucent. However, leaking margins, secondary caries, poor proximal contacts, wear of proximal surfaces, and other problems cannot be detected unless adequate radiographic contrast can be achieved. Thus, radiopacity is an important property. Radiopacity is most often imparted by adding certain glass filler particles containing heavy metal atoms, such as Ba, Sr, or Zn, and other heavy-metal/heavy-atom compounds such as YbF3, which strongly absorb x-rays. For optimal diagnostic contrast, the restoration should have a radiopacity approximately equal to that of enamel, which is about twice that of dentin. A wide range of radiopacity values have been considered to be adequate, but exceeding the radiopacity of enamel by a large degree will have the effect of obscuring radiolucent areas caused by gap formation or secondary caries.
Most important properties are improved by increased filler loading. A distribution of particle sizes is used to maximize loading (volume fraction of filler). If particle size is uniform, no matter how tightly packed the particles are, spaces will exist among them, as illustrated by the example of the void spaces in a box filled with spheres of the same size. The maximal theoretical packing fraction for close-packed spherical structures of uniform-size is approximately 74% by volume, as shown in Figure 13-7. However, if smaller particles are inserted among the larger spheres, the void space can be reduced. By extending this process, a continuous distribution of progressively smaller particles can yield maximal filler loading.
Classifications of Filled Resins
Classification by Filler Particle Size
The classification by filler particle sizes is shown in Table 13-2.
TABLE 13-2
Classification of Reinforcing Filler Particles by Size Range
Class of Filler | Particle size |
Macrofillers | 10 to 100 µm |
Small/fine fillers | 0.1 to 10 µm |
Midfillers | 1 to 10 µm |
Minifillers | 0.1 to 1 µm |
Microfillers | 0.01 to 0.1 µm (agglomerated) |
Nanofillers | 0.005 to 0.1 µm* |
Microfilled Composites.
Microfilled composites are agglomerates of 0.01- to 0.1-µm inorganic colloidal silica particles embedded in 5- to 50-µm resin filler particles. The problems of surface roughening and low translucency associated with traditional and small-particle composites can be overcome through the use of colloidal silica particles such as the inorganic filler component, with a mean particle diameter about one tenth of the wavelength of visible light (i.e., about 40 nm). Such a filler is made by a pyrolytic precipitation process where a silicon compound such as SiCl4 is burned in an oxygen/hydrogen atmosphere to form macromolecular chains of colloidal silica (see Figure 13-4) resulting in amorphous silica (colloidal, noncrystalline SiO2), which produces highly polishable esthetic composite restorations.
However, these particles, because of their extremely small size, have extremely large surface areas ranging from 50 to 400 m2 per gram. In addition, the pyrolytic process results in particle “agglomeration” into long, molecular-scale chains (see Figures 13-5 and 13-6). These nondiscrete three-dimensional chainlike networks drastically increase monomer viscosity and make clinical manipulation difficult. Filler incorporation at high enough loading to adequately reinforce the resin is also difficult with these fillers. In fact, when incorporated directly in “homogeneous” microfill composites, only about 2% by weight produces a stiff paste that is much too viscous for clinical manipulation (see Figure 13-7). However, in the manufacturing process, a resin with a very high loading (60% to 70% by weight, about 50% by volume) of silane-treated colloidal silica microfiller can be added to the monomer at a slightly elevated temperature to lower its viscosity. This is cured and then pulverized to make a filled resin powder consisting of 5- to 50-µm particles. This amorphous colloidal silica-containing resin is then used as an “organic” filler (“heterogeneous” microfill composite), which is incorporated into the monomer with additional silane-treated colloidal silica to form a workable paste. In this way the overall inorganic filler content of the final, cured composite is increased to about 50% by weight. However, if the composite particles are counted as filler particles, the filler content is closer to 80% by weight (approximately 60% by volume). Thus, the resin particles are themselves composites that contain 0.01 to 0.1 µm of amorphous silica (see Figure 13-6). A diagram representing the preparation of the filler in microfilled resins of this type is shown in Figure 13-8.
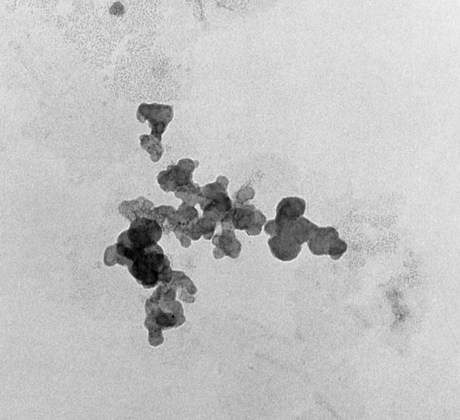
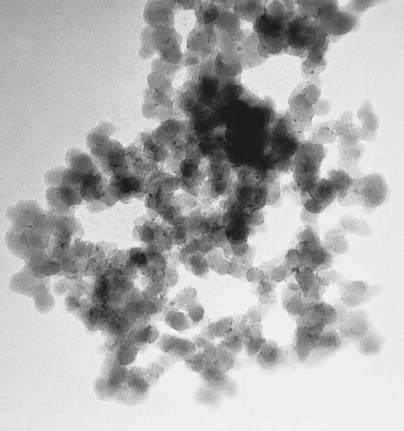
One should note that because these composite particles do not shrink when the composite is cured, a microfilled composite, despite having a much lower inorganic filler loading than the traditional or small-particle composites, will not shrink as much as expected based on the total resin volume. However, a major shortcoming of these materials is that the bond between the composite particles and the clinically cured matrix is relatively weak, facilitating wear by a chipping mechanism (Figure 13-9). Because of this deficiency, with some notable exceptions, microfilled composites are not generally suitable for use as stress-bearing surfaces.
Whereas microfilled composites are among the more highly polishable restorative composites, their physical and mechanical properties are generally inferior to those of traditional composites (Table 13-3). This is to be expected, because 40% to 80% by volume of the restorative material is made up of resin, resulting in greater water sorption, a higher coefficient of thermal expansion, and decreased elastic modulus. In addition, the weak bond of the prepolymerized particles to the clinically cured resin matrix results in decreased tensile strength, similar to that of composites with nonsilanized filler particles. In addition, in the longer term, if microfilled composites are placed in wear-prone areas, they eventually break down and wear at a rate too fast for acceptable clinical performance. If placed in areas of proximal contact, anterior tooth “drifting” may occur. The wear process has also been related to fracture propagation around the poorly bonded “organic” filler particles. Thus, diamond burs, rather than fluted tungsten-carbide burs, are recommended for trimming microfilled composites so as to minimize the risk of chipping. Microfilled composites are the resins of choice for restoring teeth with carious lesions in smooth surfaces (classes III and V) but not in stress-bearing situations (classes II and IV).
TABLE 13-3
Properties of Composite Restorative Materials
Characteristic/Property | Unfilled Acrylic | Traditional | Hybrid (Small-Particle) | Hybrid (All-Purpose) | Microfilled | Flowable Hybrid | Packable Hybrid | Enamel | Dentin |
Size (µm) | — | 8–12 | 0.5–3 | 0.4–1.0 | 0.04–0.4 | 0.6–1.0 | Fibrous | — | — |
Inorganic filler (vol%) | 0 | 60–70 | 65–77 | 60–65 | 20–59 | 30–55 | 48–67 | ||
Inorganic filler (wt%) | 0 | 70–80 | 80–90 | 75–80 | 35–67 | 40–60 | 65–81 | — | — |
Compressive strength (MPa) | 70 | 250–300 | 350–400 | 300–350 | 250–350 | — | — | 384 | 297 |
Tensile strength (MPa) | 24 | 50–65 | 75–90 | 40–50 | 30–50 | — | 40–45 | 10 | 52 |
Elastic modulus (GPa) | 2.4 | 8–15 | 15–20 | 11–15 | 3–6 | 4–8 | 3–13 | 84 | 18 |
Thermal expansion coefficient (ppm/°C) | 92.8 | 25–35 | 19–26 | 30–40 | 50–60 | — | — | — | — |
Water sorption (mg/cm2) | 1.7 | 0.5–0.7 | 0.5–0.6 | 0.5–0.7 | 1.4–1.7 | — | — | — | — |
Knoop hardness (KHN) | 15 | 55 | 50–60 | 50–60 | 25–35 | — | — | 350–430 | 68 |
Curing shrinkage (vol%) | 8–10 | — | 2–3 | 2–3 | 2–3 | 3–5 | 2–3 | — | — |
Radiopacity (mm Al) | 0.1 | 2–3 | 2–3 | 2–4 | 0.5–2 | 1–4 | 2–3 | 2 | 1 |
Hybrid Composites.
As the name implies, hybrid composites are formulated with mixed filler systems containing both microfine (0.01 to 0.1 µm) and fine (0.1 to 10 µm) particle fillers in an effort to obtain even better surface smoothness than that provided by the small-particle composites while still maintaining the desirable mechanical properties of the latter. Thus, they are a general utility class of composite that are also suitable for restoring certain high-stress-sites where esthetic considerations dominate—for example, incisal edges and small non-contact occlusal cavities. They are widely used for anterior restorations, including class IV sites (Figures 13-10 and 13-11/\).
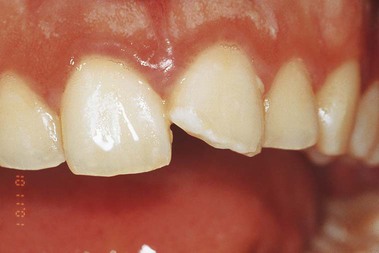
Most modern hybrid fillers consist of colloidal silica and ground particles of glasses containing heavy metals, constituting a filler content of approximately 75% to 80% by weight (see Table 13-1). The glasses have an average particle size of about 0.4 to 1.0 µm, with a trend to steadily reduce this size range as improvements are made. In a typical size distribution, 75% of the ground particles are smaller than 1.0 µm and colloidal silica represents 10% to 20% by weight of the total filler content. The smaller microfiller sizes increase the surface area, which generally increases the viscosity and requires a decrease in overall filler loading as compared with small-particle composites. A polished surface is shown in Figure 13-12.
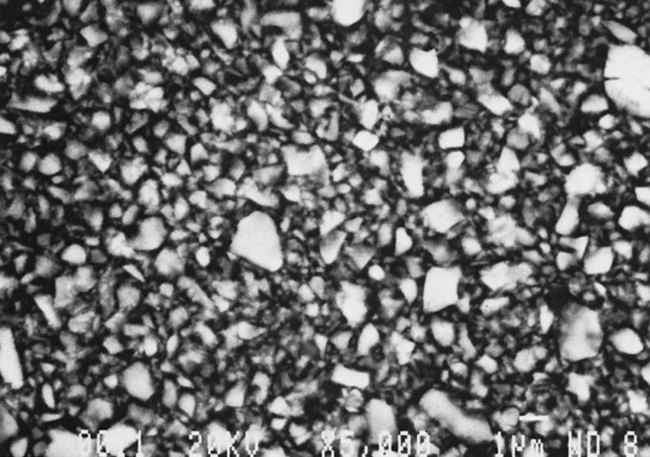
Nanofilled Composites/Nanocomposites/Nanohybrid Composites.
To combat this deficiency, larger particles of either finely ground glass or precured nanoparticle-filled resin organic filler particles (essentially the same as those found in the microfilled composites) are combined with the monomer-dispersed nanoparticles. Thus, most, if not all, of these products are more accurately designated as “hybrid” nanocomposites or nanohybrids consisting of a blend of two or more size ranges of filler particles, one or more of which is in the nanoparticle range (see Table 13-1).
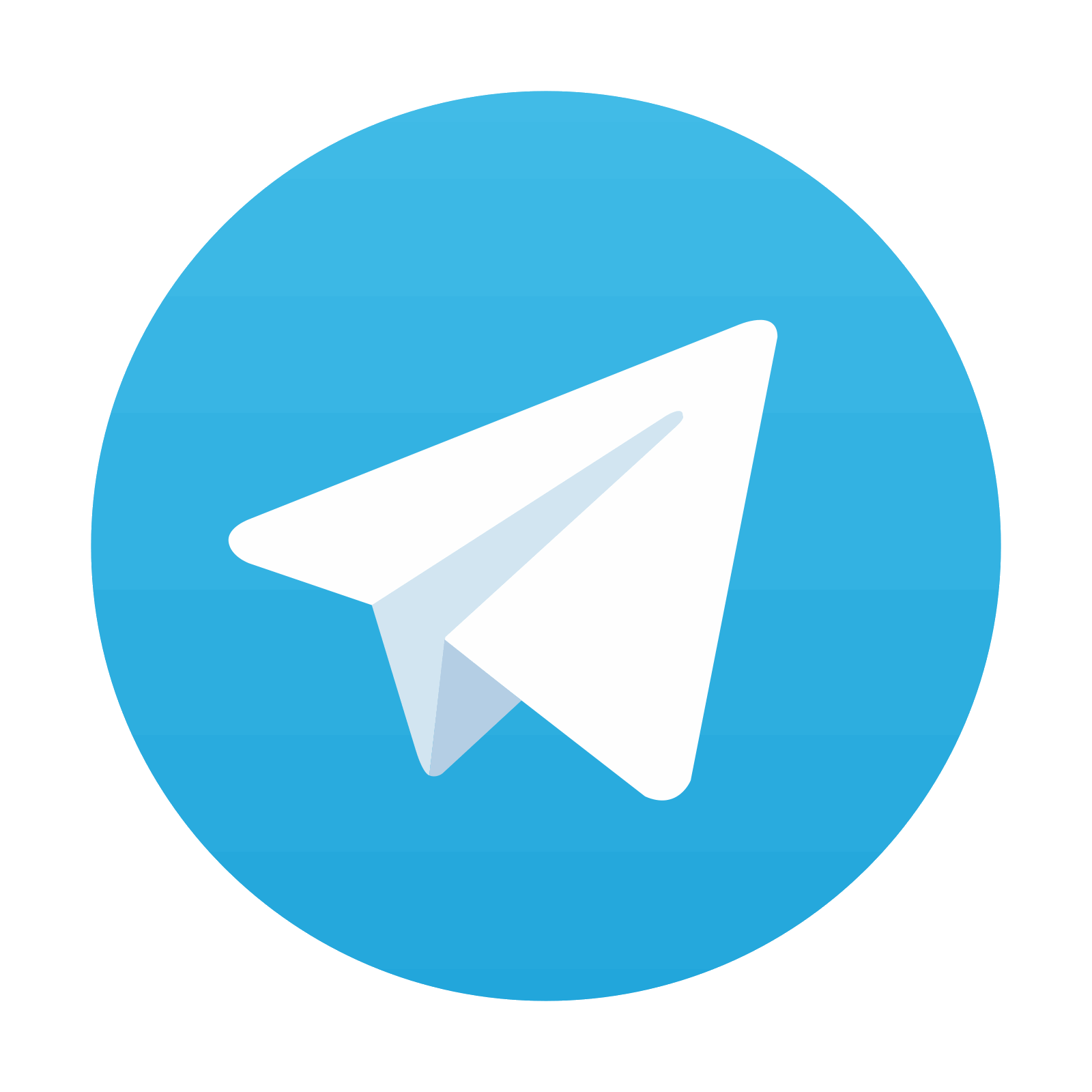
Stay updated, free dental videos. Join our Telegram channel

VIDEdental - Online dental courses
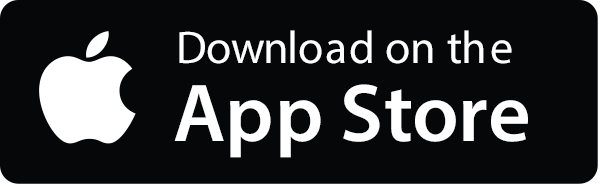
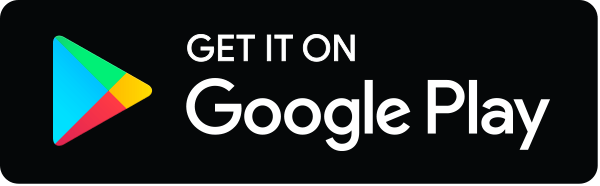