Highlights
- •
TiO 2 nanotubes were synthesized using an alkaline hydrothermal process.
- •
Nanotubes were functionalized and used to reinforce a flowable dental composite.
- •
Reinforced composites exhibited superior mechanical properties.
- •
Reinforcement had little effect on flowability, radiopacity or cytotoxicity.
- •
Nanotube-reinforced composites are promising materials for dental restorations.
Abstract
Objectives
Flowable dental composites are used as restorative materials due to their excellent esthetics and rheology. However, they suffer from inferior mechanical properties compared to conventional composites. The aim of this study was to reinforce a flowable dental composite with TiO 2 nanotubes (n-TiO 2 ) and to assess the effect of n-TiO 2 surface modifications on the mechanical properties of the reinforced composite.
Methods
n-TiO 2 were synthesized using an alkaline hydrothermal process and then functionalized with silane or methacrylic acid (MA). Nanotubes were characterized by scanning and transmission electron microscopy, X-ray diffraction, energy-dispersive X-ray spectroscopy and Fourier transform infrared spectroscopy. Commercially available flowable composite (Filtek™ Supreme Ultra Flowable Restorative, 3M ESPE) was reinforced with varying amounts of nanotubes (0–5 wt%). Flowability of the resulting composites was evaluated using a Gillmore needle method. Dynamic Young’s modulus ( E ) was measured using an ultrasonic technique. Fracture toughness ( K Ic ) was assessed using a notchless triangular prism and radiopacity was quantified. Viability of NIH/3T3 fibroblasts was evaluated following incubation on composite specimens for 24 h.
Results
Electron microscopy revealed a tubular morphology of n-TiO 2 . All reinforced composites exhibited significantly greater values of E than unreinforced composite. Composites reinforced with 3 wt% n-TiO 2 functionalized with MA exhibited the greatest values of E and K Ic . Cytotoxicity assays revealed that reinforced composites were biocompatible. Taken together, flowable composites reinforced with n-TiO 2 exhibited mechanical properties superior to those of unreinforced composite, with minimal effects on flowability and radiopacity.
Significance
n-TiO 2 -reinforced flowable composites are promising materials for use in dental restorations.
1
Introduction
Although amalgam has excellent mechanical properties and longevity, it is not esthetically pleasing. Moreover, the possible release of mercury has raised concerns regarding potential adverse health effects. As a result, focus has shifted from amalgam to resin-based composites for dental restorations . Conventional composites were introduced in dentistry more than 50 years ago. Since that time, many characteristics have been optimized, including filler size, to improve wear resistance and achieve highly polished surfaces .
More recently, flowable composites have been developed to improve handling characteristics, but mechanical properties remain inferior to those of conventional composites. It has been reported that flowable composites have 20–25% less filler content and consequently 20–30% lower modulus of elasticity . In current practice, flowable composites are used as liners, fissure sealants and class V restorative materials. The fluidity of these composites is increased by reducing the filler content or by adding modifying agents, such as surfactants. Though no particular approach for improving mechanical properties has been found to be ‘ideal’, incorporation of TiO 2 nanotubes (n-TiO 2 ) into acrylic bone cement can enhance its fracture and flexural properties without significantly altering viscosity .
Nano-sized fillers are characterized by a high surface to volume ratio. The hollow structure of nanotubes allows interlocking with the matrix on internal and external surfaces of the tubes . As a result, higher filler content can be achieved in composites, resulting in reduced polymerization shrinkage and improved mechanical properties . n-TiO 2 are typically synthesized using the alkaline hydrothermal method. This method is advantageous as it produces pure-phase nanostructures in a one-step reaction that is both reproducible and cost-effective . In terms of biomedical applications, n-TiO 2 -modified surfaces have been used successfully to improve bone regeneration by stem cells and osseointegration of implants in animal models . Moreover, nanostructured hydroxyapatite has been incorporated in root canal sealers and adhesive resins, leading to improved mechanical properties . On the other hand, nanoparticles tend to agglomerate in their native state becoming resistant to dispersion by organic solvents. Therefore, surface modification of n-TiO 2 particles is essential to prevent agglomeration and improve compatibility with resin matrices. In this regard, silane can be used to modify the surface of inorganic fillers (including TiO 2 nanoparticles) within composite materials to improve their dispersion and bonding to the resin matrix . The goal of the present study was to assess the effects of surface modification of n-TiO 2 on their ability to reinforce a commercially available flowable dental composite. Different weight percentages of n-TiO 2 (0–5%) were incorporated into Filtek™ Supreme Ultra Flowable Restorative (3M ESPE). We investigated the dynamic Young’s modulus, fracture toughness, flowability, radiopacity and cytotoxicity of the resulting materials. We hypothesized that reinforcement of a commercial flowable dental composite with n-TiO 2 will improve its mechanical properties and that surface modification of n-TiO 2 will further enhance these effects.
2
Materials and methods
2.1
Synthesis of TiO 2 nanotubes
Titania nanotubes (n-TiO 2 ) were synthesized using the alkaline hydrothermal process . Briefly, synthesis was started by dispersing 0.24 g strontium acetate powder in 30 mL of 10 M NaOH solution and stirring for 24 h. Next, 2 g of TiO 2 spherical nanoparticles (<100 nm particle size; Sigma–Aldrich, Oakville, ON, Canada) were added to the solution. The mixture was placed in a Teflon-lined stainless steel autoclave (Parr Instrument Company, Moline, IL, USA). This reaction was held at 160 °C for 20 h. The alkali-treated material was washed with deionized water and 0.1 N HCl until the pH dropped to 6.0. Finally, the resulting nanotubes were vacuum dried overnight and calcined at 400 °C for 2 h .
2.2
Functionalization of TiO 2 nanotubes
Coupling agents have functional groups to chemically link the filler and the matrix . n-TiO 2 were functionalized by coating with bifunctional coupling agents, silane or methacrylic acid (MA), as described previously . For functionalization with silane, n-TiO 2 was dispersed in 20 mL cyclohexane and 0.028 mL n -propylamine before the addition of a 10% silane mixture (7.5% 3-methacryloxypropyltrimethoxysilane, MPTMS and 2.5% n-octyltrimethoxysilane, OTMS). After 24 h under constant stirring at room temperature, the mixture was transferred to a rotary evaporator. The temperature was elevated to 95 °C for 1 h and the reaction product was dried under vacuum at 80 °C overnight .
Methacrylic acid (MA) functionalization reaction was carried out under a reflux condenser at 85 °C for 24 h. The reaction was started by dispersing 0.1 g n-TiO 2 in 35 mL of 2-propanol, after which 3 mL MA was added. The pH was adjusted to 5.0 by adding drops of 0.3 N KOH. Afterwards, the reaction product was separated by centrifugation and decantation, washed with distilled water and dried under vacuum at 80 °C overnight. The vinyl double bond of MA is known to have the ability to copolymerize, resulting in formation of nTiO 2 –PMMA composite .
2.3
Characterization of TiO 2 nanotubes
X-ray diffraction (XRD) was performed using Cu Kα radiation (30 kV, 10 mA) and D2 PHASER diffractometer (Bruker AXS, Karlsruhe, Germany) to determine the n-TiO 2 crystalline phase. The samples were prepared for XRD by gently grinding 0.1 g of the calcined n-TiO 2 using a mortar and pestle. The powder was placed evenly on a plastic XRD flat plate and positioned in the diffractometer. Data were collected at a scattering angle of 2 θ from 2° to 82° with 0.05° step size and 10°/min scan speed. 2 θ for equivalent Cu Kα radiation was calculated using Bragg’s law . Elemental analysis of n-TiO 2 was performed using a FIB/SEM LEO 1540XB microscope equipped with an energy dispersive X-ray (EDX) spectrometer (Carl Zeiss, Oberkochen, Germany).
Functionalized surfaces of n-TiO 2 were investigated by Fourier transform infrared spectroscopy (FTIR) using the KBr pellet method and a Vector 22 FT-IR Spectrometer (Bruker Optics, Ettlingen, Germany). Samples were prepared by adding 0.2 g anhydrous KBr to 2 mg of either silane- or MA-functionalized n-TiO 2 , followed by pressing at 10 tons for 10 min. Data were collected at a resolution of 4 cm −1 and wavenumbers ranging between 4000 and 1000 cm −1 .
The morphology of n-TiO 2 powder and composite fracture surfaces (following fracture toughness testing) was examined by scanning electron microscopy (SEM) (Zeiss LEO 1540XB). Samples were coated with osmium. Morphology of the n-TiO 2 was confirmed by transmission electron microscopy (TEM). n-TiO 2 was added to an Eppendorf tube containing filtered deionized water. A drop of this suspension was placed on a carbon grid and left to dry in air for 2 min then examined using a CM10 transmission electron microscope (Philips, Eindhoven, The Netherlands).
2.4
Preparation of composite samples
Flowable composite (Filtek™ Supreme Ultra Flowable Restorative) was from 3M ESPE (St. Paul, MN, USA) . The inorganic components of this composite are ytterbium trifluoride (particle sizes 0.1–5.0 μm), non-agglomerated/non-aggregated surface-modified silica fillers (20 and 75 nm), and a surface-modified aggregated zirconia/silica cluster filler (20 nm silica, 4–11 nm zirconia particles). The filler is approximately 65% by weight (46% by volume) of the material. The balance of Filtek™ flowable composite consists of resin formed by photopolymerization of the following monomers: 2,2-bis[4-(2-hydroxy-3-methacryloxypropoxy)phenyl]propane (BisGMA); tri[ethylene glycol]dimethacrylate (TEGDMA); and 2,2-bis[4-(3-methacryloxypropoxy)phenyl]propane (Procrylat).
Flowable composite specimens were reinforced by incorporating different weight percentages (0, 0.5, 1, 1.5, 2, 3, 5%) of n-TiO 2 (by manual mixing on a glass slab using a spatula) followed by light curing (550 mW/cm 2 ) for 90 s using a VALO ® LED curing light (Ultradent Products, South Jordan, UT, USA). Four groups of composites were prepared: control flowable composite (0% n-TiO 2 ); flowable composite reinforced with non-functionalized (NF) n-TiO 2 ; flowable composite reinforced with silane-functionalized n-TiO 2 ; and flowable composite reinforced with MA-functionalized n-TiO 2 . Specimen discs (5 mm diameter × 2 mm thickness) were prepared for evaluation of radiopacity and cytotoxicity, as well as measurement of dynamic Young’s modulus. Notchless triangular prisms (NTP, 6 mm × 6 mm × 6 mm × 12 mm) were prepared for testing of fracture toughness. All samples were soaked in deionized water at 37 °C for one week prior to mechanical testing.
2.5
Flowability assessment
The flowability testing method used in this study is in accord with the American Dental Association guidelines for evaluating the flowability of endodontic sealing materials . Bayne and others have used this method to evaluate the flowability of dental composites . To evaluate flowability, 0.1 mL of each composite were dispensed between two 50 mm × 50 mm glass plates (slide cover glass, Eastman Kodak, Rochester, NY, USA). These plates were placed under a constant weight (454 g) using a 1 mm diameter needle for 30 s (Gillmore needle method). As a result, the composite spread forming a disk ( Fig. 1 ). The composite discs were then subjected to light curing for 30 s. Disk diameters were determined from 10 measurements per sample, each obtained at a different orientation using a linear scale − the larger the diameter, the greater the flowability.

2.6
Mechanical testing
Dynamic Young’s moduli ( E ) of disk specimens (5 mm diameter × 2 mm thickness) were measured using a non-destructive ultrasonic system ( n = 3). In brief, this technique determines the time required for ultrasonic wave propagation through the specimen using piezoelectric crystals (lithium niobate) at a resonant frequency of 10 MHz . Data obtained using this method exhibit low coefficients of variation , permitting small sample sizes to be used.
Fracture toughness ( K Ic ) of 6 mm × 6 mm × 6 mm × 12 mm notchless triangular prism (NTP) test specimens was measured using an Instron 3345 Single Column Testing System (Instron, Norwood, MA, USA) at a crosshead speed of 0.1 mm/min. The maximum load at fracture was recorded and then K Ic was calculated using the formula described by Ruse et al. . The total test time was typically 15 min per specimen ( n = 8).
2.7
Radiopacity
Composite disk specimens ( n = 3 for each group) were placed on size 4 occlusal film (Kodak InSight dental film, F-speed) together with 3 pieces of lead and an aluminum (Al) step wedge (10-step wedge, 0.5 mm/step). The film was exposed to an X-ray beam using a Gendex GX-770 dental X-ray unit (Gendex Dental Systems, Hatfield, PA, USA) at 70 kV and 7 mA for 0.47 s. The distance between X-ray source and the film was 50 cm. Lead backing was placed under the film to minimize backscatter radiation. Following exposure, the film was developed using an automatic processor. Radiographs were scanned digitally, and radiopacity was calculated and expressed in terms of equivalent aluminum thickness (in mm) as described previously .
2.8
In vitro assessment of cytotoxicity
NIH/3T3 cells, a mouse embryonic fibroblast cell line, were obtained from the American Type Culture Collection (Manassas, VA, USA). Culture medium consisted of Dulbecco’s Modified Eagle Medium (DMEM; GIBCO ® Catalog # 11 995, Invitrogen, Burlington, ON, Canada) supplemented with 10% fetal bovine serum (FBS; GIBCO ® Catalog # 12 483) and 1% antibiotic–antimycotic solution (GIBCO ® Catalog # 14040). Composite disk specimens (5 mm diameter × 2 mm thickness) were cleaned and sterilized under low-temperature, radio-frequency glow discharge argon plasma using a PDC-32G plasma cleaner (Harrick Plasma, Ithaca, NY). For each experiment, a set of triplicate disk specimens for each group were placed in 96-well culture plates (Falcon ® Cell Culture Plates, Corning ® , Corning, NY). Cells were then seeded at the following densities: 10 × 10 3 , 20 × 10 3 and 30 × 10 3 cells/cm 2 . The plates were then incubated at 37 °C in 5% CO 2 /95% air. After 24 h, viability was assessed using 3-(4,5-dimethylthiazol-2-yl)-2,5-diphenyltetrazolium bromide (MTT) (Cell Proliferation Kit I (MTT), Roche Diagnostics, Mannheim, Germany). MTT labeling reagent was added (10 μL per well) and incubated for 4 h. Solubilizing solution (100 μL per well) was then added and left overnight. Absorbance was recorded using a Tecan Safire microplate reader (Tecan Group, Männedorf, Switzerland).
2.9
Statistical analyses
Results are presented as means ± SD or SE, as indicated. Differences among three or more groups were evaluated by one-way or two-way analysis of variance (ANOVA) followed by Tukey’s multiple comparisons tests. Straight lines were fit by linear regression using Prism 6 (GraphPad Software, La Jolla, CA, USA). Differences were accepted as statistically significant at P < 0.05.
2
Materials and methods
2.1
Synthesis of TiO 2 nanotubes
Titania nanotubes (n-TiO 2 ) were synthesized using the alkaline hydrothermal process . Briefly, synthesis was started by dispersing 0.24 g strontium acetate powder in 30 mL of 10 M NaOH solution and stirring for 24 h. Next, 2 g of TiO 2 spherical nanoparticles (<100 nm particle size; Sigma–Aldrich, Oakville, ON, Canada) were added to the solution. The mixture was placed in a Teflon-lined stainless steel autoclave (Parr Instrument Company, Moline, IL, USA). This reaction was held at 160 °C for 20 h. The alkali-treated material was washed with deionized water and 0.1 N HCl until the pH dropped to 6.0. Finally, the resulting nanotubes were vacuum dried overnight and calcined at 400 °C for 2 h .
2.2
Functionalization of TiO 2 nanotubes
Coupling agents have functional groups to chemically link the filler and the matrix . n-TiO 2 were functionalized by coating with bifunctional coupling agents, silane or methacrylic acid (MA), as described previously . For functionalization with silane, n-TiO 2 was dispersed in 20 mL cyclohexane and 0.028 mL n -propylamine before the addition of a 10% silane mixture (7.5% 3-methacryloxypropyltrimethoxysilane, MPTMS and 2.5% n-octyltrimethoxysilane, OTMS). After 24 h under constant stirring at room temperature, the mixture was transferred to a rotary evaporator. The temperature was elevated to 95 °C for 1 h and the reaction product was dried under vacuum at 80 °C overnight .
Methacrylic acid (MA) functionalization reaction was carried out under a reflux condenser at 85 °C for 24 h. The reaction was started by dispersing 0.1 g n-TiO 2 in 35 mL of 2-propanol, after which 3 mL MA was added. The pH was adjusted to 5.0 by adding drops of 0.3 N KOH. Afterwards, the reaction product was separated by centrifugation and decantation, washed with distilled water and dried under vacuum at 80 °C overnight. The vinyl double bond of MA is known to have the ability to copolymerize, resulting in formation of nTiO 2 –PMMA composite .
2.3
Characterization of TiO 2 nanotubes
X-ray diffraction (XRD) was performed using Cu Kα radiation (30 kV, 10 mA) and D2 PHASER diffractometer (Bruker AXS, Karlsruhe, Germany) to determine the n-TiO 2 crystalline phase. The samples were prepared for XRD by gently grinding 0.1 g of the calcined n-TiO 2 using a mortar and pestle. The powder was placed evenly on a plastic XRD flat plate and positioned in the diffractometer. Data were collected at a scattering angle of 2 θ from 2° to 82° with 0.05° step size and 10°/min scan speed. 2 θ for equivalent Cu Kα radiation was calculated using Bragg’s law . Elemental analysis of n-TiO 2 was performed using a FIB/SEM LEO 1540XB microscope equipped with an energy dispersive X-ray (EDX) spectrometer (Carl Zeiss, Oberkochen, Germany).
Functionalized surfaces of n-TiO 2 were investigated by Fourier transform infrared spectroscopy (FTIR) using the KBr pellet method and a Vector 22 FT-IR Spectrometer (Bruker Optics, Ettlingen, Germany). Samples were prepared by adding 0.2 g anhydrous KBr to 2 mg of either silane- or MA-functionalized n-TiO 2 , followed by pressing at 10 tons for 10 min. Data were collected at a resolution of 4 cm −1 and wavenumbers ranging between 4000 and 1000 cm −1 .
The morphology of n-TiO 2 powder and composite fracture surfaces (following fracture toughness testing) was examined by scanning electron microscopy (SEM) (Zeiss LEO 1540XB). Samples were coated with osmium. Morphology of the n-TiO 2 was confirmed by transmission electron microscopy (TEM). n-TiO 2 was added to an Eppendorf tube containing filtered deionized water. A drop of this suspension was placed on a carbon grid and left to dry in air for 2 min then examined using a CM10 transmission electron microscope (Philips, Eindhoven, The Netherlands).
2.4
Preparation of composite samples
Flowable composite (Filtek™ Supreme Ultra Flowable Restorative) was from 3M ESPE (St. Paul, MN, USA) . The inorganic components of this composite are ytterbium trifluoride (particle sizes 0.1–5.0 μm), non-agglomerated/non-aggregated surface-modified silica fillers (20 and 75 nm), and a surface-modified aggregated zirconia/silica cluster filler (20 nm silica, 4–11 nm zirconia particles). The filler is approximately 65% by weight (46% by volume) of the material. The balance of Filtek™ flowable composite consists of resin formed by photopolymerization of the following monomers: 2,2-bis[4-(2-hydroxy-3-methacryloxypropoxy)phenyl]propane (BisGMA); tri[ethylene glycol]dimethacrylate (TEGDMA); and 2,2-bis[4-(3-methacryloxypropoxy)phenyl]propane (Procrylat).
Flowable composite specimens were reinforced by incorporating different weight percentages (0, 0.5, 1, 1.5, 2, 3, 5%) of n-TiO 2 (by manual mixing on a glass slab using a spatula) followed by light curing (550 mW/cm 2 ) for 90 s using a VALO ® LED curing light (Ultradent Products, South Jordan, UT, USA). Four groups of composites were prepared: control flowable composite (0% n-TiO 2 ); flowable composite reinforced with non-functionalized (NF) n-TiO 2 ; flowable composite reinforced with silane-functionalized n-TiO 2 ; and flowable composite reinforced with MA-functionalized n-TiO 2 . Specimen discs (5 mm diameter × 2 mm thickness) were prepared for evaluation of radiopacity and cytotoxicity, as well as measurement of dynamic Young’s modulus. Notchless triangular prisms (NTP, 6 mm × 6 mm × 6 mm × 12 mm) were prepared for testing of fracture toughness. All samples were soaked in deionized water at 37 °C for one week prior to mechanical testing.
2.5
Flowability assessment
The flowability testing method used in this study is in accord with the American Dental Association guidelines for evaluating the flowability of endodontic sealing materials . Bayne and others have used this method to evaluate the flowability of dental composites . To evaluate flowability, 0.1 mL of each composite were dispensed between two 50 mm × 50 mm glass plates (slide cover glass, Eastman Kodak, Rochester, NY, USA). These plates were placed under a constant weight (454 g) using a 1 mm diameter needle for 30 s (Gillmore needle method). As a result, the composite spread forming a disk ( Fig. 1 ). The composite discs were then subjected to light curing for 30 s. Disk diameters were determined from 10 measurements per sample, each obtained at a different orientation using a linear scale − the larger the diameter, the greater the flowability.
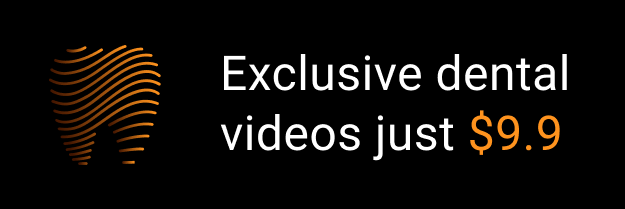