Bone grafting in reconstruction of the oral and maxillofacial region is a common procedure, with literature publication starting in the late 1800s and the earliest citations noted in the 1950s. Within the oral cavity, bone grafts are subject to wound dehiscence, infection, and graft resorption; donor site morbidity may also occur for autogenous grafts. Although autogenous bone grafts are still the gold standard, clinicians and researchers are constantly investigating other sources for grafting, including allografts, alloplasts, and osteoinductive recombinant proteins to decrease or eliminate the need for a secondary surgical site for hard tissue oral and maxillofacial reconstruction.
In the oral and maxillofacial region, cartilage repair is limited anatomically to the articular disk in the temporomandibular joint (TMJ) and auricular and nasal cartilage. When comparing bone and cartilage repair, it is well accepted that cartilaginous repair and regeneration is much more challenging than bone.
Etiopathogenesis and Causative Factors
Congenital and acquired bone defects are common in the oral and maxillofacial region. Cleft palate is the second most common congenital deformity following cardiac anomalies, and craniosynostosis occurs in 1 in 3000 live births. Other congenital defects and syndromes such as ectodermal dysplasia, Treacher Collins syndrome, hemifacial microsomia, and many others present with craniofacial bony defects that are challenging to correct or reconstruct. Other defects specific to the jaws, including maxillary and mandibular hypoplasia, are corrected with orthognathic surgery and often require bone grafting. Systemic pathologic conditions that require surgical intervention or bony reconstruction include acromegaly and ankylosing spondylitis. Acquired bone defects from trauma, tumor, infection, surgical procedures, and alveolar bone atrophy are also seen daily in our clinical practices. These bony defects usually require multiple and extensive grafting procedures, which often yield suboptimal anatomic, functional, or esthetic results.
Defects in nasal, auricular, or TMJ articular cartilage are generally caused by traumatic injury, infection, pathology, or degeneration. Difficulties with cartilaginous repair after injury are well known. Cartilage has a limited capacity for self-repair because of its minimal vascular supply and low cellular density. In addition, full thickness articular cartilage injuries that affect the underlying bone often heal by the formation of fibrocartilage, which can lead to rapid degradation upon further injury. Large defects usually require autologous cartilaginous grafting for nasal and auricular defects, or total joint replacement without an articular disk for the TMJ area, which also often yield suboptimal results.
Anatomic Considerations
In performing surgery to repair or reconstruct bone and cartilage in the oral and maxillofacial region, the surgeon must be familiar with normal anatomy of both the injury and the donor sites if autologous tissue grafting will be used. Patients who have been edentulous for many years or have congenitally missing teeth have significant alveolar bone deficiencies. Since the deficiency increases with time, early intervention with bony reconstruction may decrease the continued resorption associated with prolonged edentulism. Another important consideration is the importance of vascularity on wound healing and repair. This is apparent when evaluating the success of bone grafts, where vascularity determines the incorporation of the graft to the recipient bed. When using local flaps, they also must have adequate vascularity for survival. Incisions must be placed in easily manipulated locations and orientations so that the flaps can cover bone grafts without tension to protect the graft from contamination or infection.
For cartilage regeneration, anatomic differences between tissues such as the ear and nose are apparent when compared to load-bearing joints such as the knee and TMJ. Even anatomic differences between load-bearing joints may affect their ability to perform specific functions, especially since the TMJ is composed of fibroelastic cartilage, whereas the knee joint is composed of articular or hyaline cartilage. Also, in the treatment of arthritis, significant differences exist between the TMJ and other joints. In some orthopedic situations, possible treatments include fusion, which is never an acceptable option for the TMJ.
Anatomic Considerations
In performing surgery to repair or reconstruct bone and cartilage in the oral and maxillofacial region, the surgeon must be familiar with normal anatomy of both the injury and the donor sites if autologous tissue grafting will be used. Patients who have been edentulous for many years or have congenitally missing teeth have significant alveolar bone deficiencies. Since the deficiency increases with time, early intervention with bony reconstruction may decrease the continued resorption associated with prolonged edentulism. Another important consideration is the importance of vascularity on wound healing and repair. This is apparent when evaluating the success of bone grafts, where vascularity determines the incorporation of the graft to the recipient bed. When using local flaps, they also must have adequate vascularity for survival. Incisions must be placed in easily manipulated locations and orientations so that the flaps can cover bone grafts without tension to protect the graft from contamination or infection.
For cartilage regeneration, anatomic differences between tissues such as the ear and nose are apparent when compared to load-bearing joints such as the knee and TMJ. Even anatomic differences between load-bearing joints may affect their ability to perform specific functions, especially since the TMJ is composed of fibroelastic cartilage, whereas the knee joint is composed of articular or hyaline cartilage. Also, in the treatment of arthritis, significant differences exist between the TMJ and other joints. In some orthopedic situations, possible treatments include fusion, which is never an acceptable option for the TMJ.
Diagnostic Studies
In evaluating bone repair or planning bone reconstruction, adequate radiographs are required. For most of the oral and maxillofacial region, panoramic radiographs and CT scans are a necessity. MRIs are also important in evaluating the anatomy of the articular disk and surrounding soft tissues and detecting potential disk dislocation, with or without reduction upon mouth opening. Bone scans may also be used to rule out continued pathologic activity for certain disease processes. Being able to evaluate the defect and its planned reconstruction in three dimensions is important in restoring esthetics, form, and function to the face or jaws. The advent of three-dimensional (3D) models as tools to aid in complex maxillofacial reconstruction offers more detailed presurgical planning and identification of potential complications.
Treatment and Reconstructive Goals
The goals of repair and reconstruction are to recapture the normal form, function, and esthetics of the bone and cartilage that existed before injury. Specific for a bone graft, several principles must be followed: (1) remove diseased tissue; (2) establish functional occlusion; (3) restore or maintain adequate vascularity; (4) provide a base for prosthetic reconstruction, with or without dental implants; (5) maintain space; (6) provide soft tissue coverage; (7) maintain stability; and (8) prevent infection.
Normal Healing
Normal Bone Healing (Repair)
Bone healing undergoes the same three stages seen with soft tissue healing: inflammatory, fibroplastic, and remodeling. The inflammatory stage begins immediately after injury and lasts up to 5 days. Initially, vasoconstriction allows a blood clot to form, and then cells with inflammatory and phagocytic properties are attracted to the wound site to decrease debris and bacteria accumulation. The fibroplastic stage involves fibroblasts that produce fibrin, which increases wound strength over the next 2 to 3 weeks. Increased vascularity with new budding capillaries is also important in this stage. Increased blood flow brings cells and various factors to induce fibrinolysis as the fibrin network continues to mature. Finally, the remodeling stage involves reorganization and strengthening of the wound, but with significant wound contraction to close the gap.
Extraction sockets heal in a similar manner, but the inflammatory stage takes approximately 2 weeks to form the blood clot, break down debris, and deposit osteoid. The fibroplastic stage occurs during the next 2 weeks, with epithelialization of the socket and formation of woven bone. The remodeling stage lasts 4 to 6 months, during which cortical and trabecular woven bone is resorbed and replaced by lamellar bone. Healing of fractures also follows these three stages, but the process is further classified as healing by primary or secondary intention. Primary intention occurs when the fracture edges are precisely placed without a gap between the segments, which often requires rigid internal fixation and immobilization to promote more rapid healing and decrease scarring and risk of infection. Healing by secondary intention occurs with bone avulsion or imperfect anatomic reduction with a residual space between the two bony edges. In these situations, more collagen deposition is required to bridge the gap, resulting in callus formation with the involvement of periosteal and overlying soft tissue responses. If healing continues with stability, lack of infection, and adequate vascularization, remodeling will occur and the callus will calcify and become bone. The process of creeping substitution occurs here, where the gradual formation of osteoid tissue across the defect slowly matures to normal bone. It is important to understand that healing of a fracture requires specific responses in the bone marrow, cortex, periosteum, and overlying soft tissues. If these do not occur, a fibrous union will result.
Normal Cartilage Healing (Repair)
The need for cartilage repair usually results from damage caused by trauma, pathologic condition, or arthritis. Although repairing damaged cartilaginous tissue is difficult enough in healthy states, repair in the presence of disease such as arthritis or autoimmune disorders poses a unique challenge. Cartilage healing and repair is significantly more limited than in bone because of its minimal potential for self-regeneration. Even though cartilage is a metabolically active tissue, it lacks inherent blood and lymphatic vessels. Inflammation does not occur and progenitor cells from the blood or bone marrow cannot access damaged tissue. Therefore cartilage is unable to repair or regenerate itself.
Current evidence suggests that additional cartilage on articular surfaces can only form during the first 3 months of postnatal life. Damage to articular cartilage where cartilage and bone are in direct contact extends from the articular surface to the subchondral bone. Damage is accompanied by bleeding into the defect, which activates the normal bone healing process, and inflammation triggers hematoma formation. As the repair process continues with the fibroplastic stage, where fibroblasts begin to increase wound strength, the resultant fibrous tissue is not consistent with the structural and functional properties of articular cartilage. In partial thickness defects where the injury is contained within the articular cartilage, blood and cells cannot access the injury site. In these cartilaginous injuries, the defect remains. This significant challenge with cartilage healing has clinicians and researchers searching for techniques to successfully regenerate damaged cartilage.
Specific Treatment and Techniques
Bone Grafting
Bone grafting is a common technique used to treat damaged or missing bone in orthopedic and oral and maxillofacial surgery. Historically, autogenous bone is the gold standard for critical size defects in the craniofacial region. Autogenous bone possesses all the important biological processes: osteogenesis, the formation of new bone from transplantation of osteocompetent cells; osteoinduction, formation of new bone from the differentiation and stimulation of undifferentiated mesenchymal stem cells; and osteoconduction, formation of new bone along a scaffold from the host osteocompetent cells at the recipient site. Many donor sites are available for autogenous bone grafts, including extraoral sites such as the iliac crest, tibia, rib, and calvarium, and intraoral sites such as the ramus, chin, and tuberosity. Extraoral sites provide a larger volume of bone than intraoral sites, but they carry greater donor site morbidity. Cortical bone, as harvested from the calvarium, has less resorption but a slower revascularization rate than cancellous bone from the iliac crest or tibia. Cancellous bone, also called trabecular bone, provides pluripotent or osteogenic precursor cells and more rapid revascularization of the graft. Alternatively, use of intraoral donor sites decreases the need for hospitalization and lowers cost, but these sites cannot be used to reconstruct large maxillofacial defects.
Since both intraoral and extraoral autogenous bone grafts are limited in quantity and require a second surgical site, the use of bone substitutes continues to increase. Allografts are bone grafts from a donor of the same species. They are usually sterilized by gamma irradiation or ethylene oxide to eliminate disease transmission ; however, the risk of interindividual infection is still a concern with the use of allografts. Although a large number of studies in the orthopedic and oral and maxillofacial literature document favorable outcomes with the use of allografts, only osteoconductive and potential osteoinductive properties are documented.
Other alternatives to autogenous bone grafts are alloplastic bone substitutes and xenografts. Xenografts are bone grafts from another species, usually bovine, for oral and maxillofacial surgery. Xenografts are deproteinized to remove all organic material, and are often combined with ceramics such as hydroxyapatite. Ceramics also are used as bone substitutes, including various combinations of hydroxyapatite and β-tricalcium phosphate (β-TCP), bioactive glasses, porous hydroxyapatite, calcium sulfate, and calcium carbonate. These materials have no bioactive proteins and possess only osteoconductive properties. As a result of the lack of inductive properties, alloplast uses are limited to small space-maintaining defects in oral and maxillofacial surgery, based on defect volume, location, and host factors.
Hard Tissue Engineering
In recent years, a new era in reconstructive surgery has begun with the application of tissue engineering, including the use of cells, scaffolds, and growth factors to improve bone healing and regeneration. When engineering a bony construct with this triad of components, it may be possible to create an ideal, easily available, and highly reliable bone graft material with no donor site morbidity and a low risk of infection or antigenicity. Osteogenic or osteoinductive signals could be preserved, the construct could be easily manipulated but remain stable, and vascular supply could be maintained. In evaluating a complex craniofacial defect, a 3D scaffold that can withstand pressures from the overlying soft tissue is necessary to adequately reconstruct the missing hard tissue. These scaffolds must maintain space to allow for tissue regeneration; provide an environment for cell attachment and possibly proliferation, differentiation, or both; and endure functional loading that supports bone growth. The scaffold must be degraded, metabolized, or excreted easily, and be cost-effective when processed to fit complex defects. The scaffold also should provide an interface with cell receptors and extracellular matrix to facilitate the functional interaction that allows normal cellular functions to occur. To date, poly-L-lactic acid (PLLA) and poly-L-glycolic acid (PLGA) are commonly used synthetic scaffolds in bone tissue engineering because they are biologically inert and safe to use in humans. In vivo animal studies have shown the ability of these scaffolds to regenerate bone in defects of either critical or non-critical size when incorporated with recombinant growth factors. Many other synthetic as well as naturally occurring scaffolds have been successfully used for bone repair and regeneration, including PLGA/polyethylene glycol (PEG), calcium phosphate, collagen, alginate, silk, polycaprolactone, poly(propylene fumarate), and others.
In addition to the use of synthetic or naturally occurring scaffolds, their surface properties can be altered, including texture, roughness, hydrophobicity, charge, and chemical composition, to enhance the interaction with cells for improved cell signaling, differentiation, and function. Indeed, marrow stromal cells (MSCs) can be induced to convert to an osteogenic phenotype when seated on a porous, dense scaffold. Using the scaffold to create a more favorable microenvironment for interaction with local cells and factors can improve the osteoinductivity of the construct, which is important when scaffolds are used as carriers for temporal and spatial release of growth factors and proteins.
Growth factors and proteins that reside in the extracellular matrix play crucial roles in the bone healing process. Factors such as bone morphogenetic protein-2 (BMP-2), basic fibroblast growth factor (bFGF), platelet-derived growth factor (PDGF), transforming growth factor-β (TGF-β), and vascular endothelial growth factor (VEGF) have shown positive effects in promoting fracture healing. BMPs are now well known to induce expression of osteoblast markers and stimulate bone formation in vivo, and are the focus of much of the bone regeneration studies in both translational research and clinical practice. BMPs make up a large portion of the TGF-β superfamily, which regulates key steps in the differentiation, proliferation, and morphogenetic processes of bone and cartilage. BMPs are among the most potent regulators of osteoblast differentiation and BMP types 2, 4, 6, and 7 all have osteoinductive properties in vivo. BMP-2 released from various carriers has been shown to completely regenerate calvarial defects in the rodent model. In humans, BMP-2 recombinant protein can regenerate mandibular continuity and cleft palate defects, and augment maxillary sinuses, with outcomes that are comparable to those of autogenous particulate bone and marrow. These results have led the U.S. Food and Drug Administration (FDA) to approve rhBMP-2 on an absorbable collagen sponge for use in orthopedic surgery for spinal fusion and non-union of tibia fractures, and in oral and maxillofacial surgery for sinus augmentation and localized alveolar ridge defects.
As bone regeneration strategies become more attainable and realistic, the importance of vascularity is apparent. Formation of new vessels and revascularization of a bone graft is crucial to successful regeneration. VEGF is the most potent and widely used regulator of vascularization, with important effects on osteoblasts and osteoclasts during bone repair. Osteoblasts express VEGF receptors, and impaired VEGF-receptor signaling could lead to decreased recruitment and differentiation of osteoblasts. Animal studies have shown the ability of VEGF to enhance BMP-2 effects in critical size defects and BMP-2 has been shown to induce osteoblastic differentiation and bone formation that expresses VEGF. To improve bone regeneration, other combinations of growth factors have also been investigated to more closely mimic natural temporal and spatial expression. Chitosan-based scaffolds using VEGF pre-encapsulated in alginate microspheres allowed the delayed release of VEGF, several days after PDGF was released into the defect. Although PDGF increased bone formation over the scaffold alone, the addition of VEGF enhanced both new vessel formation and bone regeneration. Furthermore, sequential release of factors such as VEGF and PDGF are more effective at promoting angiogenesis than simultaneous release.
Soft tissue wound-healing effects of PDGF are well established, especially in diabetics with chronic non-healing ulcers on a lower extremity. PDGF has important functions in mitogenesis, angiogenesis, and recruitment of fibroblasts and osteoblasts to assist in wound healing. In animal studies, PDGF induced bone regeneration in calvarial defects when implanted on a PLLA scaffold. PDGF has also been shown to improve periodontal defects, which led to FDA approval of PDGF on a tricalcium phosphate carrier for periodontal intrabony defects.
bFGF plays a role in cell proliferation, motility, differentiation, mitogenesis, wound healing, tissue repair, and angiogenesis. Its role in alveolar bone repair and healing mandibular and long bone fractures is well established. Recent animal studies have shown preliminary success using bFGF to repair large osteochondral defects, where the regenerated cartilage demonstrated a hyaline-like appearance. Difficulty with cartilage repair and regeneration has been previously mentioned in this chapter, and will be discussed in more detail in a later section.
TGF-β has been studied for decades for its potential role in bone regeneration and repair. It has chemotactic and mitogenic properties important in promoting osteoblast differentiation and inhibiting osteoclastic bone resorption. TGF-β also functions in connective tissue repair and formation of vessels, demonstrating bone-specific properties. It is not as potent as BMP-2, a member of the larger TGF-β superfamily. Therefore, much of the research in bone regeneration has focused on BMP-2 as previously discussed.
IGFs are also mitogens for osteoblasts and osteoblast precursors to stimulate differentiation and bone formation. IGF-1 has been shown to improve bone healing in both healthy and diabetic animals and to increase bone contact when coated on implants in combination with TGF-β.
In evaluating the ideal growth factor to regenerate bone, the goal of clinicians and researchers is to recapture the normal physiologic process of bone healing and repair. This goal encourages the use of growth factor combinations or more complex scaffolds to release specific factors in a sequential or prolonged fashion. Studies evaluating sustained release of BMP-2 to increase the bioactivity, and lead to improved osteoblastic differentiation in vitro and bone formation in vivo. Combinations such as VEGF plus BMP-2, BMP-7 plus PDGF, PDGF plus VEGF, PDGF plus IGF-1, and TGF-β plus IGF-1 have all demonstrated enhanced bone healing or regeneration.
In addition to prolonging the release and combining growth factors to improve bone regeneration, the use of cells such as mesenchymal stem cells, alone, transduced with growth factors, or implanted directly with growth factors, is currently under intense investigation. MSCs can be acquired from multiple autologous sources, including iliac crest, tibia, and sternal bone marrow; periosteum; adipose tissue; synovium; skin; pericytes; umbilical cord; peripheral blood; dental pulp; periodontal ligament; deciduous teeth; and apical papillae. Under appropriate conditions, including osteogenic media (ascorbic acid, β-glycerophosphate, dexamethasone, fetal bovine serum) or treatment with osteoinductive factors such as BMP-2, TGF-β, and IGF-1, MSCs can differentiate into osteoblasts and form bone. Indeed, animal models of bone marrow stromal cells (BMSCs) with hydroxyapatite/tricalcium phosphate (HA/TCP) or calcium alginate scaffolds significantly induce bone regeneration, better than autologous bone grafts.
When recombinant growth factors are delivered, the initial high concentration is not maintained at the site for long periods and therefore limits the amount of bone formation. Gene therapy helps to overcome this limitation, where MSCs can be engineered to express osteoinductive growth factors to regenerate bony defects. This indirect, or ex vivo, gene therapy approach, where the cells are genetically modified outside the body and then reimplanted to deliver factors to a localized defect, has successfully regenerated long bone and craniofacial defects. Initial studies used MSCs that were transduced with BMP-2 and then incorporated into a collagen scaffold and implanted into segmental defects in the rat. Since then, other studies used cells from periosteum, fat, and muscle with both viral and non-viral vectors to obtain acceptable bony regeneration. In vivo gene therapy can also be performed, where a gene is directly injected using vectors within a matrix, such as fibrin glue, alginate, or collagen. This technique is simpler and more cost-effective than ex vivo gene therapy because there are no autologous cells or in vitro expansion required. Many in vivo gene therapy studies have successfully regenerated bony defects in small animals when various BMP genes are transferred. However, larger animal models have not yet provided similarly consistent results.
The combination of cells, scaffolds, and differentiation factors holds great promise as an implantable construct for 3D tissue engineering. Most likely, these cocktails will differ in each clinical situation, based on the number of factors required, timing of release, structural support required by the scaffold, cell type and differentiation potential, local environment, and type of pathology or disease. Even though the task seems quite complicated, considerable progress has been made in the field of tissue engineering for craniofacial bone regeneration, including the maxillary sinus, calvarium, and palate. Although significantly more research is needed before these techniques can replace current bone grafting procedures, human case reports demonstrate regeneration of large bony defects with combinations of growth factors, scaffolds, and MSCs.
Cartilage Regeneration
Because cartilage healing and repair is extremely limited after injury, reconstruction of ear and nose defects is often performed with cartilaginous transplantation. However, anatomic nasal septal and auricular donor sites are small and long-term results for cartilage grafting are still unknown. So research has focused on cartilage regeneration using gene therapy and engineering principles. Even more challenging are defects in a joint because the roles of a normal anatomic and functional articular surface are more complex. As discussed earlier, tissue engineering and gene therapy have been successful in bone regeneration both in animal models and clinical studies, but progress in gene therapy for cartilage regeneration has been slower and more difficult to achieve. In situ regeneration is based on the concept of introducing undifferentiated MSCs to the area of injury via holes drilled through the cartilage and into the subchondral bone. Accessing the bone marrow will attract the MSCs and the blood clot formed in the initial healing stages, providing the appropriate environment for chondrogenic differentiation. Clinically, some studies have shown favorable functional results similar to cell-based therapy with this microfracture technique in humans, even though the regenerated cartilage was not histologically identical to the original. Furthermore, factors present in the normal blood clot possess chemotactic properties for MSCs, which have potentially favorable effects for the microfracture technique. However, this process is not usually consistent with articular cartilage formation because it is more similar to normal bone formation.
Autologous chondrocytes can be harvested, grown in vitro, and injected or implanted into cartilaginous defects or areas of damage. Similar to osteogenic differentiation in vitro, chondrogenic differentiation requires a specific set of supplements in culture, including dexamethasone, TGF-β, ascorbic acid, chondroitin sulfate, serum withdrawal, or multilayer culture system. In orthopedic surgery, autologous chondrocytes have been used either alone or on a 3D carrier to treat defects in the knee and improve pain from osteoarthritis. However, the disadvantages of harvesting autologous chondrocytes are similar to those of harvesting autologous bone and bone marrow, including donor site morbidity. Some additional disadvantages of using autologous chondrocytes are dedifferentiation and loss of potential redifferentiation with increased expansion in vitro, and lack of similarities in growth and differentiation with chondrocytes from different anatomic locations.
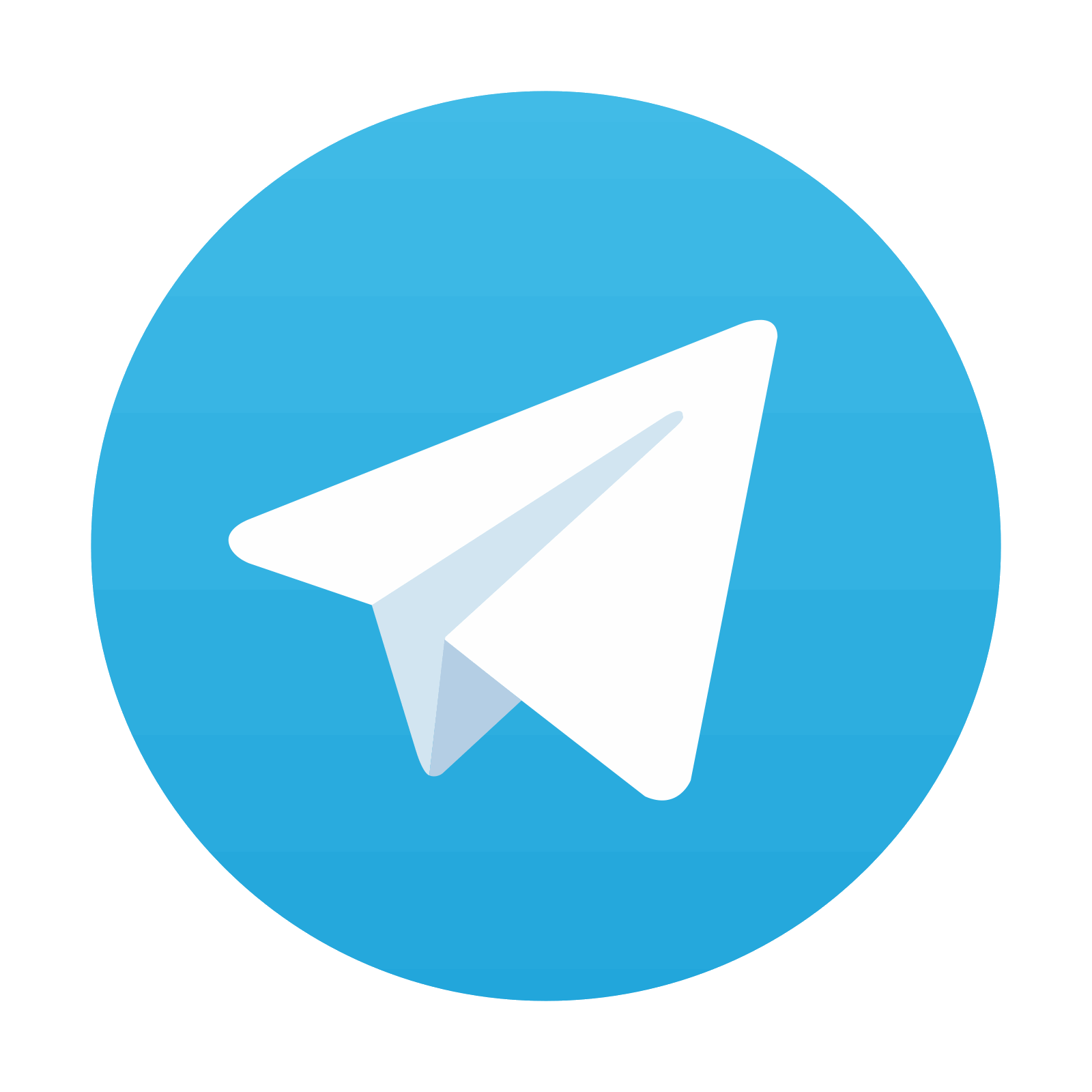
Stay updated, free dental videos. Join our Telegram channel

VIDEdental - Online dental courses
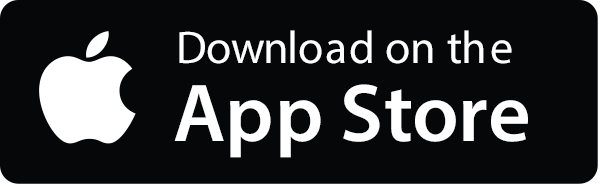
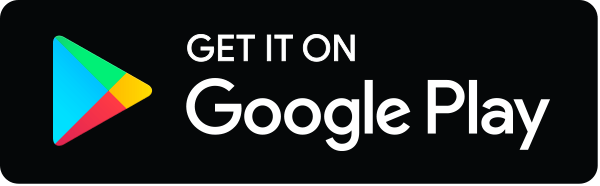