Graphical abstract

Highlights
- •
Niobium silicate particles were produced by sol-gel route and used as filler dor dental composites
- •
The addition of niobium silicate particles did not interfere on the physicomechanical properties of developed composites
- •
The biological properties were enhanced for niobium silica-containing composite resins
- •
Increased cell viability and mineral deposition were observed for the composite resins with niobium silicate addition.
Abstract
Objectives
This study aimed to evaluate the influence of niobium silicate (SiNb) particles in the physicomechanical and biological properties of an experimental composite resin.
Methods
The SiNb particles were incorporated (50 wt%) into a polymeric matrix formulated with 70 wt% Bisphenol A-Glycidyl Methacrylate and 30 wt% Triethylene Glycol Dimethacrylate to formulate an experimental composite resin. A control group was formulated with barium silicate glass (SiBa) as filler for the same polymeric matrix. The composite resins were tested for their refractive index, polymerization kinetics, flexural strength, radiopacity, softening in solvent, pH, cytotoxicity and mineral deposition.
Results
The SiBa group presented refractive index results between 1.50 and 1.52 and the SiNb between 1.43−1.45. No statistically significant difference in the degree of conversion, flexural strength, and softening in solvent was observed between different groups. Radiopacity was lower for SiNb, while the addition of these particles increased cell viability. The pH was increased for all groups after immersion. The mineral deposition analysis resulted in increased deposition above specimens after the immersion in SBF.
Significance
Niobium silicate particles may be used as an alternative inorganic filler to achieve an adequate balance between physical-chemical and biological properties for the development of bioactive composite resins.
1
Introduction
The development of composite resins with therapeutic activity may be an alternative to increase the potential of restorative materials to reduce secondary caries [ , ]. The remineralization of the tooth/material interface by bioactive materials is studied, and bioactive fillers may be used as remineralizing agents inside the polymeric matrix. Bioactive inorganic fillers such as calcium phosphates [ ] and bioactive glasses have been studied [ ] for this purpose. The addition of bioactive fillers, however, affects the mechanical stability of these materials due to the lack of adequate organic/inorganic interaction [ , ].
Silicates have been used as inorganic fillers in dental composites as they are able to adequately interact with the matrix maintaining mechanical and optical properties of materials [ ]. This interaction is generated by the presence of coupling agents, mainly the silanes, that easily bond the silica-containing particles to the polymeric matrix [ , ] This reaction happens trough the condensation of silanols on the particle surface and free-radical reaction in the resin monomers maintaining a strong and stable bonding in the particle/matrix interface. The adequate polymerization jointly with the stress dissipation throughout the particle-silane-polymer interface results in reliable materials for restorative procedures [ ] and should be guaranteed when bioactive fillers were used.
High-silica content fillers could be modified with bioactive components to enhance the biological properties of these particles. Niobium has been studied in the biomedical field due to its ability to promote the remineralization of hard tissues [ ]. Niobium oxides were studied previously as bioactive fillers for dental composites [ , , , , ]. The interaction between these particles and the polymeric matrix may be jeopardized by the absence of chemical interaction on the surface of the oxides as well as by the higher mismatch between the light refraction of these oxides when compared to the polymeric matrix [ , ]. The bonding between niobium to silicate compounds has been studied [ , ], showing that niobium could be bonded to silicate-based structures leading to chemically stable particles. The combination of silicate properties and niobium bioactivity in niobium silicate particles may be an alternative to the development of bioactive composite resins with enhanced properties. Based on this, the aim of this study was to evaluate the influence of the synthesized particles on the physicomechanical and biological properties of an experimental composite resin.
2
Materials and methods
2.1
Synthesis of niobium silicate
Niobium silicate particles were synthesized by the sol-gel route. Briefly, Tetraethylorthosilicate (TEOS – Si(OC 2 H 5 ) 4 – Aldrich Chemical; St Louis, MO, USA) was used as silica precursor, and niobium pentachloride (NbCl 5 – CBMM, Araxá, MG, Brazil) was used as an inorganic modifier. Water: Teos molar ratio was set at 10:1 while Nb:Si molar ratio was set at 1:9. After heat treatment, particles were grounded in an analytical mill (M 20 Universal mill- IKA, Campinas, Sp, Brazil) at 28,000 rpm. Milled particles were dispersed in absolute ethanol and sieved to controlling particle size distribution. The structure of the particles was evaluated by x-ray diffraction (X’PertPRO, PANalytical MPD, Netherlands) and Fourier-transform infrared spectroscopy (VERTEX 70, Bruker Optics, Ettingen, Germany). The morphology of the particles was analyzed by scanning electron microscopy (JSM-6060, Jeol, Akishima, Japan), laser diffraction (Mastersizer Malvern PANalytical, Almelo, Netherlands), and nitrogen adsorption (Quantachrome Instruments Corporate Headquarters, USA). Glassy structured particles were produced with a mean particle size of 2.054 μm with a specific surface area of 616.96m 2 /g. The characterization of synthesized particles is shown on the supplementary material ( Fig. 1 ).

2.2
Formulation of experimental composite resins
Composite resins were formulated with bisphenol A-glycidyl methacrylate (BisGMA – 70 wt%) and triethylene glycol dimethacrylate (TEGDMA – 30 wt%). Monomers were weighed and mixed in a sonication bath for 8 min. As the photoinitiator system, the trimethyl benzoyl-diphenylphosphine oxide (TPO – 1 mol%) and 0.01 wt% butylated hydroxytoluene (BHT). All reagents were purchased by Aldrich Chemical, St Louis, MO, USA.
Niobium silicate was used as filler in 50 wt% concentration to produce the SiNb group. Before the application of niobium silicate in the composite resin, particles were silanized with an acetone solution of 3-(trimethoxysilyl)propyl methacrylate (Merck KGaA, Darmstadt, Germany) at 95 wt% acetone concentration. A control group (SiBa) was produced using barium borosilicate glass in the same concentration (Esstech-Essington, Pennsylvania, USA) with a mean particle size of 4.58 μm. Colloidal silica (Aerosil 200- Evonik, Hanau, Germany) was used in both groups in 1.5 wt% to adjust the handling of the experimental composites.
2.3
Experimental composite resin properties
2.3.1
Refractive index
The refractive index for the experimental composite resins was analyzed by a spectral ellipsometer (Sopra GES-5E, Texas, USA) in a spectral range between 320 and 480 nm. Unpolymerized SiNb and SiBa composite resins were evaluated (n = 1). A filler-free resin was analyzed, as well. The amplitude of the refractive index between the spectral range was used and compared to the filler-free resin.
2.3.2
Degree of conversion and polymerization kinetics
The degree of conversion was analyzed in a spectrometer (FTIR – VERTEX 70, Bruker Optics, Ettingen, Germany) equipped with a reflectance device (ATR) by Fourier Transformed Infrared Spectroscopy (FTIR). Unpolymerized resins (n = 3) were placed in the ATR crystal in polyvinylsiloxane molds measuring 4 mm diameter x 1 mm height. Samples were polymerized with a multiwavelength light-emitting diode (Valo Cordless, Ultradent, UT, USA) at 1000 mW/cm 2 for 40 s in a standardized distance of 1 mm between the sample and the light-curing unit. The DC of the composite resins was determined by the ratio of the absorbance peak of aliphatic carbon-carbon double bonds (1640 cm −1 ) to the peak of aromatic double bonds (1610 cm −1 ), an internal standard.
For polymerization kinetics measurement, the same apparatus was used. Samples (n = 3) were placed above the ATR crystal and polymerized for 40 s. The measurements were performed from the moment the photoactivation started until its end in standardized parameters of scanning range (4000–800 cm −1 ), resolution (4 cm −1 ), and scanning time (40 s). The data were calculated based on the peak height in the 1640cm −1 and 1610 cm −1 as a function of time. The data was submitted to a sigmoidal curve fitting in a linear regression with Sigma Plot 12.0 for Windows (Systat Software Inc, San Jose, CA, USA). The degree of conversion and the polymerization rate was plotted over time, and descriptive analysis was performed.
2.3.3
Flexural strength
Flexural strength was measured according to ISO 4049 [ ]. Five rectangular specimens (n = 10) with 25 mm x 2 mm x 2 mm were prepared for each group in a metallic mold. Polymerization was performed in 2 windows for 20 s on each side of the specimen. Specimens were stored in distilled water at 37 °C for 24 h before the tests. Flexural strength was determined with a three-point test at a crosshead speed of 0.75 mm/min in a mechanical testing machine (EZ-SX – Shimadzu, Kyoto, Japan) until the specimens fractured. Flexural strength was calculated from the following equation:
where F is the maximum load exerted on the specimen, Ɩ is the distance (mm) between the supports ± 0.01 mm, b is the width (mm) of the specimen immediately before testing, and h is the height (mm) of the specimen measured with a digital caliper immediately before testing.
2.3.4
Cytotoxicity
Primary gingival fibroblasts were used to test the effect of experimental composites on cell behavior. Cells were cultivated in Dulbecco’s Modified Eagle Medium (DMEM) supplemented with 100 U/mL penicillin and 10% of FBS. Cells were cultured at 37 °C, and under 5% CO 2 / 95% air atmosphere.The medium was changed every 2–3 days. Before cell culture, samples (n = 3–4 mm diameter × 1 mm height) were immersed in 1 mL of supplemented DMEM and kept in a 37 °C environment for 24 h. For cell treatment, gingival fibroblasts (5 × 10 3 ) were seeded in 96-well plates, and after 24 h, each well was treated with 100 μl of DMEM medium conditioned with composite resin specimens. Cell treatments were performed in triplicate. After 72 h in culture, cells were fixed and stained with 50 μl SRB 0.4% (Sigma Aldrich, St. Louis, EUA). Quantification of viable cells was performed for absorbance at 560 nm in a Microplate Spectrophotometer (Multiskan™ GO, Thermo Fisher Scientific, USA). Medium without treatment was used as a control to normalize the percentage of viable cells.
2.3.5
Radiopacity
Radiopacity was tested according to ISO 4049 [ ]. Samples (n = 5–6 mm diameter x 1 mm height) were exposed to x-ray during 0.8 s at a standardized distance of 400 mm. The X-ray source (Dabi Atlante model Spectro 70X) was operated with a tungsten anode at 70 kV, and 8 mA. Samples were placed in phosphorous plates next to an aluminum step wedge. A digital system (VistaScan- Durr Dental, Bissingen- Germany) was used to obtain the images. The pixel density in the images was measured in a standardized area in an image software (ImageJ, NIH, Maryland, USA) in the samples and the aluminum step wedge. The pixel density values were converted to mmAl according to the pixel density in each image.
2.3.6
Softening solvent
Specimens (n = 3) measuring 4 mm diameter x 1 mm height were prepared in polyvinylsiloxane molds and photoactivated for 20 s on each side. Discs were polished before an initial Knoop microhardness measurement (KHN1) in a Knoop Hardness Tester (HMV 2, Shimadzu, Japan). Three indentations were performed in each specimen with a 10 g load for 5 s. Specimens were then immersed in 70% ethanol solution for 2 h, as described previously [ , ], and a new KHN measurement was performed (KHN2). The differences between KHN1 and KHN2 were used to calculate de ΔKHN% for softening solvent analysis.
2.3.7
pH
Samples (n = 3) measuring 6 mm diameter x 1 mm height were immersed in distilled water for pH analysis in a digital pHmeter (DM 22- Digimed, São Paulo, Brazil). The initial pH of 5 mL of distilled water was initially measured, and after sample immersion, the pH was measured at 30 min, 1, 2, 3, 4, 24 h, 48 h, 72 h, 7, 14, 21 and 28 days. The water was not changed during the experiment.
2.3.8
Mineral deposition
For mineral deposition analysis, samples were produced measuring 4 mm diameter and 1 mm height. The experimental composite resins were immersed in simulated body fluid (SBF) [ ] for 7 and 14 days at 37 °C. After the determined time points, Raman spectroscopy (Senterra, Bruker Optics, Ettingen, Germany) was used to determine the amount of phosphate on the sample’s surface based on the PO 4 3− intensity at the 960 cm -1 region. The surfaces of the analyzed samples were imaged using scanning electron microscopy (JSM-6060, Jeol, Akishima, Japan).
2.4
Statistical analysis
A descriptive analysis of the characterization of niobium silicate particles was performed. The amplitude was used to describe refractive index data. The normality of data was assessed by Shapiro-Wilk. Student’s T-Test for independent samples was used to compare differences between groups in the degree of conversion, flexural strength, cytotoxicity, pH, KHN1, and %ΔKHN. The differences between KHN1 and KHN in each experimental group were assessed by the Student’s T-Test for dependent samples. Descriptive analysis was performed for polymerization kinetics and for mineral deposition results obtained by Raman spectroscopy and SEM. All tests were performed at a 5% significance level.
3
Results
No statistical difference in DC was observed between different groups. The polymerization kinetics of experimental resins is shown in Fig. 1 . The increase in the degree of conversion was slower ( Fig. 1 A), and the maximum polymerization rate ( Fig. 1 B) was reduced for SiBa group when compared to SiNb. The gel point was reached after 5.86 s for SiNb and 7.60 s for SiBa. Increased gingival fibroblasts viability was observed for SiNb composites, as shown in Table 1 . While SiBa analysis resulted in 82.51% of viable cells, the SiNb group presented 102.06% of viable cells (p < 0.05).
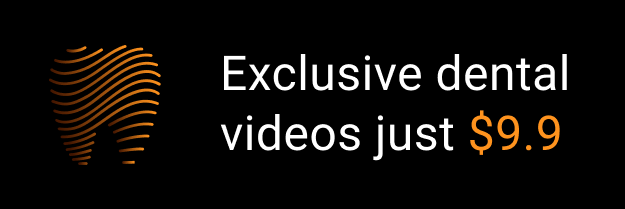