Abstract
Objectives
This study evaluated the effect of surface treatments on the bond strength of fluorcanasite and lithium disilicate glass-ceramics, with the possibility of eliminating HF etching of these ceramics.
Methods
Fifteen blocks of an experimental fluorcanasite and a lithium disilicate glass-ceramic (IPS e.max CAD ® ) were assigned to one of the following three surface treatments: (1) machined with 60 μm finish, (2) machined and grit blasted, (3) machined and HF etched. The ceramic blocks were duplicated in composite resin (Spectrum ® ) and cemented together with a resin luting agent (Variolink II ® ). Thirty microbars per group (1.0 × 1.0 × 20 mm) were obtained and subjected to a tensile force at a crosshead speed of 0.5 mm/min using a universal testing machine until failure. The mode of failure was determined using scanning electron microscopy. The appropriate bonding procedure was assessed for durability by storing in water at 100 °C for 24 h. Statistical analyses were performed with ANOVA and Tukey’s test ( P < 0.05).
Results
Machining alone significantly increased the bond strength (MPa) of the fluorcanasite (27.79 ± 6.94) compared to the lithium disilicate (13.57 ± 4.52) ( P < 0.05). HF etching resulted in the lowest bond strength (8.79 ± 2.06) for the fluorcanasite but the highest for the lithium disilicate (24.76 ± 9.38). Regarding durability, the machined fluorcanasite (15.24 ± 5.46) demonstrated significantly higher bond strength than the machined and HF etched lithium disilicate (12.28 ± 3.30).
Significance
The fitting surface of the fluorcanasite glass-ceramic should retain the machined finish and be directly treated with silane. The use of HF acid is contraindicated.
1
Introduction
Chain silicates, or inosilicates, are polymeric crystals in which single or multiple chains of silica tetrahedra form the structural backbone. In the late 1970s, Beall demonstrated that glass-ceramics based on modified chain silicate compositions (enstatite, potassium fluorrichterite and canasite) have a particularly high fracture toughness (3–5 MPa m 1/2 ) and bending strength (200–300 MPa). Fluorcanasite is a synthetic double chain silicate glass-ceramic displaying a combination of high flexural strength and fracture toughness in comparison with currently available resin-bonded glass-ceramic restorative systems and is a potential material for all-ceramic restorations. In addition, fluorcanasite, unlike many high strength dental ceramics, has a surface that could be bonded with an adhesive composite resin luting agent via a silane coupling agent.
Success with resin-bonded all-ceramic restorations is highly dependent on obtaining a durable and reliable bond, which has to integrate all parts of the system into one coherent structure. This bond is usually created by: (1) micromechanical retention by hydrofluoric acid etching and/or grit blasting, and; (2) chemical bonding by a silane coupling agent . Etching the inner surface of a restoration with hydrofluoric acid followed by the application of a silane coupling agent is a well known and recommended method to increase the bond strength . However, previous studies have challenged this protocol. Hooshmand et al. and Aida et al. found that the hydrofluoric acid etching stage could be eliminated for the bonding procedure whereas Sorensen et al. reported that the use of a silane coupling agent was of no significant benefit. Shimada et al. reported that hydrofluoric acid etching glass-ceramics adversely affects ceramic bonding and is probably not necessary for clinical applications. Glass-ceramics with a fine crystalline structure such as fluorcanasite may not benefit from hydrofluoric acid etching. Other researchers have demonstrated that the new generation of ceramic primers can strongly couple to machinable glass-ceramics without prior gritblasting or hydrofluoric acid etching of the ceramic surface .
There are possibly three good reasons why it would be desirable to remove the hydrofluoric acid etching step from the procedure: (1) hydrofluoric acid is a highly toxic chemical, representing a potentially serious health hazard ; (2) it has been reported that hydrofluoric acid etching of silica-based ceramics produces insoluble silica-fluoride salts, which can remain as by-products on the surface . If not removed, these by-products can interfere with the bond strength to the resin; (3) its elimination from the bonding procedure would be highly advantageous, but would only be possible if the silane bond can be shown to be adequate .
Various investigations have demonstrated that using adhesive composite resin cements increases the fracture resistance of glass-ceramic restorations, provides high retention, improves marginal adaptation and prevents microleakage by penetrating surface flaws and irregularities and inhibiting crack propagation . Fracture resistance of the ceramic–resin bond is controlled primarily by the microstructure and surface treatment of the ceramic . Therefore, it is essential that an optimal bonding protocol is developed. Because fluorcanasite is a chain silicate glass-ceramic, it is hypothesized that it is possible to achieve a reliable bond using a silane coupling agent and resin cement. Due to the fine grain, acicular microstructure of fluorcanasite, it may be possible to eliminate the hydrofluoric acid etching stage from the cementation procedure.
2
Materials and methods
2.1
Ceramic materials
Two CAD/CAM machinable glass-ceramic core materials were employed in this study; an experimental fluorcanasite glass-ceramic (University of Sheffield) and a commercial lithium disilicate glass-ceramic (e.max CAD ® , batch number JO8179, Ivoclar Vivadent AG, Schaan, Liechtenstein).
2.2
Surface preparation
Four different surface treatments were performed on disc specimens of the fluorcanasite and lithium disilicate glass-ceramic:
- •
Polished to 1 μm finish with 400–1200-grit wet silicon carbide paper, then 3 and 1 μm diamond polishing paste using a polishing machine (Buehler Metaserv, UK).
- •
Machined finish using a 60 μm diamond bur (Henry Schein, Germany).
- •
Machined and grit blasted with 50 μm aluminium oxide particles (MicroEtcher, Danville Engineering, San Ramon, CA).
- •
Machined and etched with hydrofluoric acid (HF) (Ultradent Porcelain Etch 9.5% Buffered, Ultradent Products, South Jordan, UT) for 1 min, then rinsed and air dried for 1 min.
A surface roughness profile was determined for each of the groups using a profilometer (Mitutoyo Surftest 301, Mitutoyo America Corp, Aurora, IL). A diamond stylus (5 μm radius) was used under a constant measuring force of 3.9 N. The instrument was calibrated using a standard reference specimen, and then set to travel at a speed of 0.1 mm/s with a range of 600 μm during testing. The roughness of the specimen was analyzed by performing two passes of the profilometer, with one pass at a 90° angle to the other. Ten recordings per specimen ( n = 3) in each surface treatment group were obtained.
Following completion of the profilometric evaluation, SEM analysis was performed to ascertain the effects of the different surface treatments on the microstructure of the core materials. The specimens were gold coated with a sputter coater (Evaporation unit, Edwards, UK), mounted on coded brass stubs and examined using scanning electron microscopy (Philips XL-20).
2.3
Microtensile bond strength testing
Fifteen 1 × 1 × 1 cm blocks were prepared from the fluorcanasite and the lithium disilicate glass-ceramics. The specimens were polished with 400-grit through to 1200-grit wet silicon carbide paper using a polishing machine (Buehler Metaserv, UK). Following this, the ceramic blocks were ultrasonically cleaned (Biosonic UC300, Whaledent, Altstätten, Switzerland) in distilled water for 5 min to remove any contamination from the silicon carbide papers. Each ceramic block was duplicated in composite resin (Spectrum TPH, batch no. 0506003114, Dentsply DeTrey GmbH, Konstanz, Germany) with the same dimensions using a mould made of a polyvinylsiloxane impression material (Aquasil, Dentsply DeTrey GmbH, Konstanz, Germany). Incremental layers (2 mm) of composite resin were condensed into the mould under a standardized load of 40 N, polymerized for 20 s (LCU, Bayer Dental, Leuerkusen) and repeated until the mould was full. One composite resin block was constructed for each ceramic block.
The 15 blocks of each ceramic were randomly assigned to three groups which received the following surface treatments:
- •
Machined finish using a 60 μm diamond bur (Henry Schein, Germany). This surface finish was used to simulate the machining process of the CAD/CAM technology.
- •
Machined finish and grit blasting with 50 μm aluminium oxide particles (MicroEtcher, Danville Engineering, San Ramon, CA).
- •
Machined finish and HF acid (Ultradent Porcelain Etch 9.5% Buffered, Ultradent Products, South Jordan, UT) applied for 1 min, rinsed for 1 min and air dried for 1 min.
The silane coupling agent (Monobond-S, batch no. J14325, Ivoclar Vivadent AG, Schaan, Liechtenstein) was then applied to each group with a brush, left undisturbed for 1 min and then dried with an air stream. The ceramic and composite resin blocks were then joined as pairs using a composite resin luting system (Variolink II ® , batch no. J17818, Ivoclar Vivadent AG, Schaan, Liechtenstein) according to the manufacturer’s instructions and light polymerized with a standard halogen light (LCU, Bayer Dental, Leuerkusen) for four 40 s periods at right angles to each other. The specimens were stored in distilled water for 24 h.
Using a water-cooled diamond blade with a slow speed cutting saw (Isomet, Buehler, Lake Bluff, IL, USA) each block was longitudinally cut into a series of 1 mm thick slabs. The sectioning continued until 1 mm remained to keep the specimen in a fixed position. The ceramic–composite block was then rotated 90° and the procedure repeated. Twelve to fourteen bars approximately 1.0 mm 2 in cross-section were obtained from each block. The peripheral slices were disregarded in case the results could be influenced by either excess or insufficient amount of resin cement at the interface.
Each microbar was glued with cyanoacrylate (Zapit, CA, USA) to a jig designed to transmit purely tensile forces when mounted on a universal loading machine (Lloyds LRX tensometer, Lloyds Instruments, UK). Bending forces were avoided by gluing specimens in the most parallel position possible and in contact with the jig. The tensile load (100 N) was applied at a crosshead speed of 0.5 mm/min until failure. The load at failure in Newtons was recorded, and the fragments of the specimen were carefully removed from the fixture with a scalpel blade. The cross-sectional area at the site of fracture was measured to the nearest 0.01 mm with a digital calliper (Mitutoyo, Tokyo, Japan) in order to calculate the bond strength at failure in MPa.
The microtensile bond strength values were calculated using the formula σ = L / A where L is the load at failure (Newtons) and A is the adhesive area (mm 2 ) and expressed in MPa. Thirty microbars from each group were tested for microtensile bond strength.
The fractured surfaces were examined by optical microscopy (Bausch and Lomb, USA) and scanning electron microscopy (Philips XL-20) to determine the type of failure, which was classified as adhesive (A), cohesive (C) or mixed (M) within any of the substrates or interfaces. The fractures surfaces were gold coated (Evaporation unit, Edwards, UK) prior to examination.
2.4
Durability of the bond
The surface treatment methods which afforded the highest microtensile bond strengths were also assessed for bond durability. Thirty microbars of the fluorcanasite and lithium disilicate glass-ceramic, with surface treatments of machined and machined plus HF etching respectively, were fabricated as described before. The specimens were then stored in distilled water at 100 °C (boiling water) for 24 h using an extraction apparatus. After a 30 min drying period, the microbars were subjected to the same microtensile bond strength test.
2.5
Statistical analysis
The data were analyzed using ANOVA with Tukey’s multiple comparison tests. The software programme used was SPSS for Windows, Version 14.0, SPSS Inc., Chicago, IL. The results were considered significant for P < 0.05.
2
Materials and methods
2.1
Ceramic materials
Two CAD/CAM machinable glass-ceramic core materials were employed in this study; an experimental fluorcanasite glass-ceramic (University of Sheffield) and a commercial lithium disilicate glass-ceramic (e.max CAD ® , batch number JO8179, Ivoclar Vivadent AG, Schaan, Liechtenstein).
2.2
Surface preparation
Four different surface treatments were performed on disc specimens of the fluorcanasite and lithium disilicate glass-ceramic:
- •
Polished to 1 μm finish with 400–1200-grit wet silicon carbide paper, then 3 and 1 μm diamond polishing paste using a polishing machine (Buehler Metaserv, UK).
- •
Machined finish using a 60 μm diamond bur (Henry Schein, Germany).
- •
Machined and grit blasted with 50 μm aluminium oxide particles (MicroEtcher, Danville Engineering, San Ramon, CA).
- •
Machined and etched with hydrofluoric acid (HF) (Ultradent Porcelain Etch 9.5% Buffered, Ultradent Products, South Jordan, UT) for 1 min, then rinsed and air dried for 1 min.
A surface roughness profile was determined for each of the groups using a profilometer (Mitutoyo Surftest 301, Mitutoyo America Corp, Aurora, IL). A diamond stylus (5 μm radius) was used under a constant measuring force of 3.9 N. The instrument was calibrated using a standard reference specimen, and then set to travel at a speed of 0.1 mm/s with a range of 600 μm during testing. The roughness of the specimen was analyzed by performing two passes of the profilometer, with one pass at a 90° angle to the other. Ten recordings per specimen ( n = 3) in each surface treatment group were obtained.
Following completion of the profilometric evaluation, SEM analysis was performed to ascertain the effects of the different surface treatments on the microstructure of the core materials. The specimens were gold coated with a sputter coater (Evaporation unit, Edwards, UK), mounted on coded brass stubs and examined using scanning electron microscopy (Philips XL-20).
2.3
Microtensile bond strength testing
Fifteen 1 × 1 × 1 cm blocks were prepared from the fluorcanasite and the lithium disilicate glass-ceramics. The specimens were polished with 400-grit through to 1200-grit wet silicon carbide paper using a polishing machine (Buehler Metaserv, UK). Following this, the ceramic blocks were ultrasonically cleaned (Biosonic UC300, Whaledent, Altstätten, Switzerland) in distilled water for 5 min to remove any contamination from the silicon carbide papers. Each ceramic block was duplicated in composite resin (Spectrum TPH, batch no. 0506003114, Dentsply DeTrey GmbH, Konstanz, Germany) with the same dimensions using a mould made of a polyvinylsiloxane impression material (Aquasil, Dentsply DeTrey GmbH, Konstanz, Germany). Incremental layers (2 mm) of composite resin were condensed into the mould under a standardized load of 40 N, polymerized for 20 s (LCU, Bayer Dental, Leuerkusen) and repeated until the mould was full. One composite resin block was constructed for each ceramic block.
The 15 blocks of each ceramic were randomly assigned to three groups which received the following surface treatments:
- •
Machined finish using a 60 μm diamond bur (Henry Schein, Germany). This surface finish was used to simulate the machining process of the CAD/CAM technology.
- •
Machined finish and grit blasting with 50 μm aluminium oxide particles (MicroEtcher, Danville Engineering, San Ramon, CA).
- •
Machined finish and HF acid (Ultradent Porcelain Etch 9.5% Buffered, Ultradent Products, South Jordan, UT) applied for 1 min, rinsed for 1 min and air dried for 1 min.
The silane coupling agent (Monobond-S, batch no. J14325, Ivoclar Vivadent AG, Schaan, Liechtenstein) was then applied to each group with a brush, left undisturbed for 1 min and then dried with an air stream. The ceramic and composite resin blocks were then joined as pairs using a composite resin luting system (Variolink II ® , batch no. J17818, Ivoclar Vivadent AG, Schaan, Liechtenstein) according to the manufacturer’s instructions and light polymerized with a standard halogen light (LCU, Bayer Dental, Leuerkusen) for four 40 s periods at right angles to each other. The specimens were stored in distilled water for 24 h.
Using a water-cooled diamond blade with a slow speed cutting saw (Isomet, Buehler, Lake Bluff, IL, USA) each block was longitudinally cut into a series of 1 mm thick slabs. The sectioning continued until 1 mm remained to keep the specimen in a fixed position. The ceramic–composite block was then rotated 90° and the procedure repeated. Twelve to fourteen bars approximately 1.0 mm 2 in cross-section were obtained from each block. The peripheral slices were disregarded in case the results could be influenced by either excess or insufficient amount of resin cement at the interface.
Each microbar was glued with cyanoacrylate (Zapit, CA, USA) to a jig designed to transmit purely tensile forces when mounted on a universal loading machine (Lloyds LRX tensometer, Lloyds Instruments, UK). Bending forces were avoided by gluing specimens in the most parallel position possible and in contact with the jig. The tensile load (100 N) was applied at a crosshead speed of 0.5 mm/min until failure. The load at failure in Newtons was recorded, and the fragments of the specimen were carefully removed from the fixture with a scalpel blade. The cross-sectional area at the site of fracture was measured to the nearest 0.01 mm with a digital calliper (Mitutoyo, Tokyo, Japan) in order to calculate the bond strength at failure in MPa.
The microtensile bond strength values were calculated using the formula σ = L / A where L is the load at failure (Newtons) and A is the adhesive area (mm 2 ) and expressed in MPa. Thirty microbars from each group were tested for microtensile bond strength.
The fractured surfaces were examined by optical microscopy (Bausch and Lomb, USA) and scanning electron microscopy (Philips XL-20) to determine the type of failure, which was classified as adhesive (A), cohesive (C) or mixed (M) within any of the substrates or interfaces. The fractures surfaces were gold coated (Evaporation unit, Edwards, UK) prior to examination.
2.4
Durability of the bond
The surface treatment methods which afforded the highest microtensile bond strengths were also assessed for bond durability. Thirty microbars of the fluorcanasite and lithium disilicate glass-ceramic, with surface treatments of machined and machined plus HF etching respectively, were fabricated as described before. The specimens were then stored in distilled water at 100 °C (boiling water) for 24 h using an extraction apparatus. After a 30 min drying period, the microbars were subjected to the same microtensile bond strength test.
2.5
Statistical analysis
The data were analyzed using ANOVA with Tukey’s multiple comparison tests. The software programme used was SPSS for Windows, Version 14.0, SPSS Inc., Chicago, IL. The results were considered significant for P < 0.05.
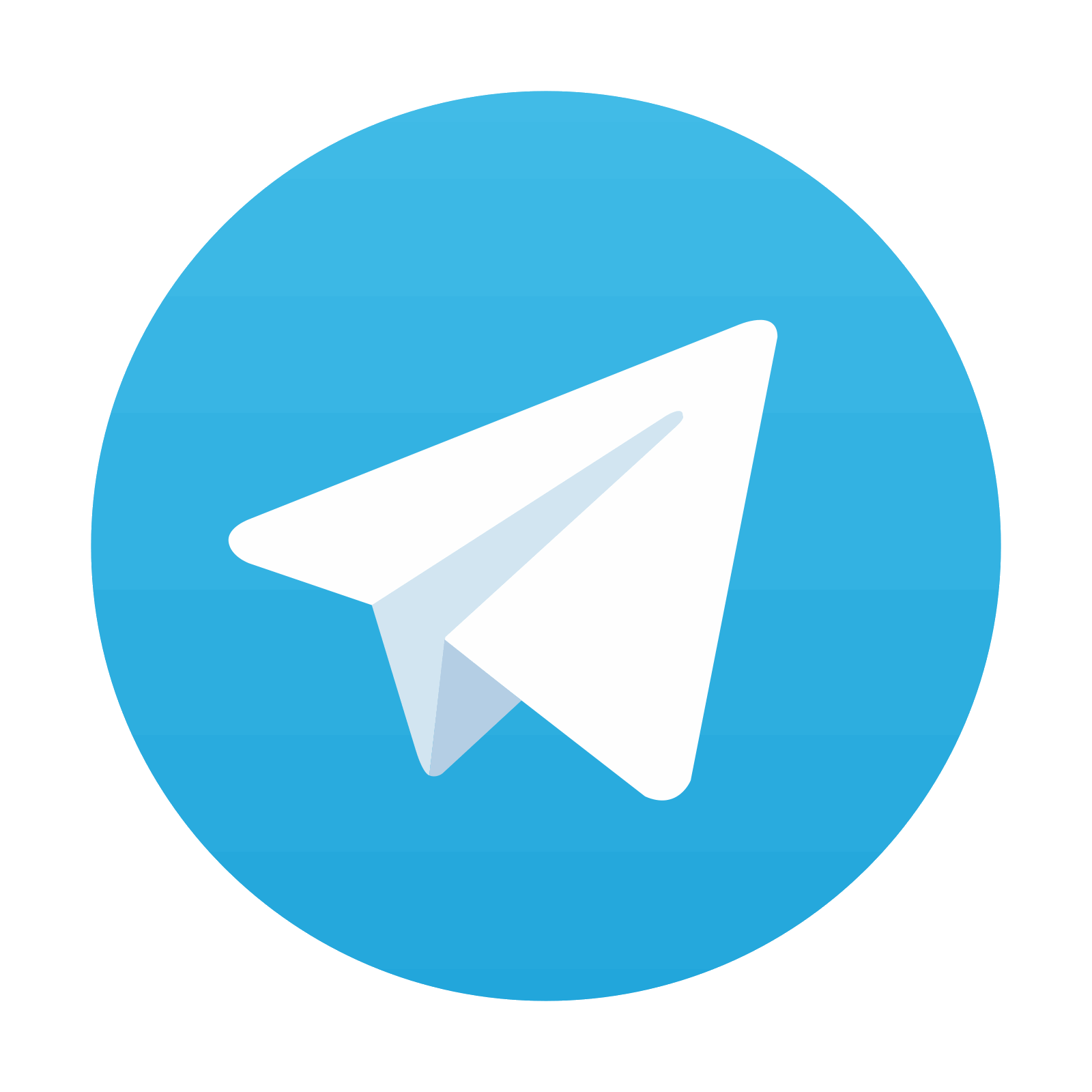
Stay updated, free dental videos. Join our Telegram channel

VIDEdental - Online dental courses
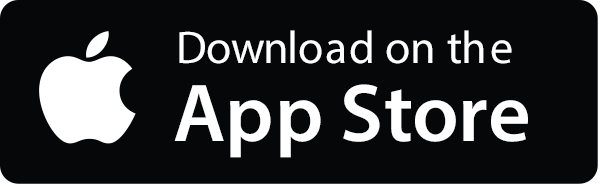
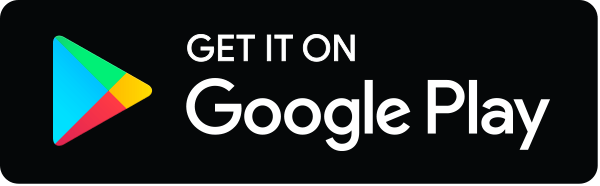