Abstract
Objective
The result of this research was to develop a quantum mechanically based QSAR model for polymer flexural modulus from structural features of small oligomers of the polymers. The final model was to have both explanatory power and be a reasonably accurate screening tool for new materials.
Methods
A quantitative structure–activity relationship (QSAR) was developed using the CODESSA program from quantum mechanical information provided by the AM1 semiempirical method, as implemented in AMPAC.
Results
A four-descriptor correlation equation with R 2 = 0.91, also satisfying our other statistical criteria. A tetramer was determined to be a sufficient simulator for the polymer chain. The descriptors in the model show that rigidity of the monomer, electrostatic interactions and branching are the most important contributors to the flexural modulus value for a particular system.
Significance
The QSAR model we have developed here is conceptually satisfying for flexural, and provides an easily usable tool for rational biomaterials design.
1
Introduction
Inorgancially filled polymer composites have been used as dental restorative materials with great success for more than half a century, since Bowen first patented resins based on Bis-GMA in the 1960s . The lack of mercury in composite restoratives is one significant advantage over traditional amalgam materials, minimizing damage to human health and the environment. Moreover, composites’ color similarity to dentin is also a remarkable aesthetic improvement . However, amalgam still remains a widely used restorative material in dental clinics, and more than 70 million amalgam restorations are placed every year in the United States . The primary reason that polymer composites have not substantially replaced amalgam is due to amalgam’s better longevity and sustainability . Another barrier to universal acceptance of polymer composites result from toxic interactions of unpolymerized leachates with metabolic systems in the body . Massive effort has been applied to solving these problems, with notable but limited success .
The clinical performance of a dental restorative material depends on many interacting factors, and those relationships have proven to be difficult to understand. One of the targets of dental materials development has been high mechanical strength, assuming that higher values lead to better long-term clinical outcomes. Dental polymer composites consist of three main components: (1) the resin , consisting of the monomer, photo-initiators and other additives; (2) the filler , containing inorganic reinforcing materials such as silica, quartz or glass; and (3) coupling agents , that produce covalent linkages between matrix and filler . The primary contributor to mechanical strength in the cured composite is the reinforcing filler, but it is clear that the coupling agents and polymer matrix are also important to mechanical performance . Coupling agents are typically long chains of covalently bonded atoms that terminate in a polymerizable group that is chemically compatible with the monomers in the matrix resin. Thus, these linkages are covalent, and it is reasonable to assume that the resin matrix fails long before these bonds would break from mechanical forces. Based on these reasons, improvement of the mechanical toughness of the matrix resin system is the most effective way to increase the restorative’s performance and improve clinical outcomes.
The polymer component must exhibit high resistance to deformation, since teeth are regularly under substantial mechanical stress, and flexural strength has traditionally been a key indicator used to rank materials for this application . As such, one goal of dental restorative polymer design has been to produce materials with high flexural strength, in the hope that such materials will perform well when combined into restorative materials. Modulus is one of the mechanical properties that demonstrate a material’s resistance against deformation under load. The flexural modulus (FM), in particular, measures this behavior under simple beam loading. Shown in Fig. 1 is the three-point bend test geometry, the most common test for polymer flexural modulus, as standardized in U.S. ASTM D790 . In this experiment, the sample beam is under compressive stress at the concave surface and tensile stress at the convex surface, and the flexural modulus is expressed as the ratio between the stress and deflection. While results can be obtained rapidly and reproducibly from this experiment, the synthesis, characterization, and formulation of materials for testing are time-consuming and expensive. Like other mechanical properties, values for the FM depend on a variety of factors including the polymer’s molecular structure, sample shape/dimensions, processing methods, testing conditions, and others.

There are a potentially enormous number of imaginable structural variations on just the commonly used methacrylate backbone, each producing different properties. Testing even a small set of candidates with no a priori method to select promising candidates is simply prohibitive. Specifically this paper will explore the relationship between the FM of the polymer matrix and its chemical structure. We here describe a quantum mechanically based quantitative structure–activity relationship model (QSAR) that predicts the flexural modulus of homopolymers based on information encoded in the molecular structures on small polymer segments. While not treating the composite that is ultimately deployed, this model directly addresses the factor most amenable to modification and measurement related to clinical composite performance, the flexural modulus.
2
Method
2.1
Training set selection
The QM-QSAR procedure develops a mathematical model by correlating experimental results for a target property (in this case flexural modulus) with discrete numerical and chemically representative indexes of information extracted from the actual structure of a simulator molecule. Obviously this demands a series of controlled experiments in which all the factors that potentially affecting the target property would be fixed, except molecular structures. Unfortunately there is no such systematic data in the literature. Therefore we must select data in such a manner as to eliminate as many variables as possible, while retaining a reasonable number of data points of sufficient structural variation. We began by obtaining literature values for experimentally determined FM limited by the following criteria:
-
Polymer composition. The polymers we chose were required to be homopolymers or alternating co-polymers as neat resins, i.e. containing no additives other than an initiation system. Additives may introduce effects on polymer behavior that is beyond the scope of molecular modeling to address.
-
Standard conventional processing. The polymers must be conventionally processed, and the flexural modulus values be measured on molded samples. Polymers that were kneaded , non-conventionally molded , annealed , or aged are excluded. Admittedly many other factors during polymer processing also play significant roles in the measured FM. For instance, atactic polystyrene has a different flexural modulus when injection molded than when compression molded . Additionally, melting temperature ( T g ), injection speed, molding temperature, die geometry, and other factors all influence the measured flexural modulus value .
-
Experimental method. The FM must have been determined by the ASTM D790 standard, or by following a scheme that complies with that standard, eliminating quite a few variables. ASTM D790 defines the shape of the specimen, span-to-depth ratio, strain rate, testing humidity, and testing temperature.
-
Elemental composition. The polymers contain C, H, O, and N only. This still encompasses a vast array of polymers, including almost all of those currently deployed as dental restorative materials. Although halogen-containing polymers were considered initially, only four polymers were found to satisfy the first three criteria. As a result they are not included in the final model.
Tightening these criteria any further reduces the number of available data points so far that no sensible, statistically valid models would be obtainable. This limitation will produce “noise” in the final results; but, the magnitude can be quantified and the result of the outcome qualitatively explained. Table 1 lists the 29 polymers satisfying these criteria that were located in the NIMS PoLyInfo database . If multiple values were available for a particular polymer, an averaged value has been used and that value is shown in Table 1 as the experimental value. We retained 25 of the original 29 materials for the training set. A group of four polymers were excluded as outliers, and the rationale for that will be further discussed below. For extensibility of the model, we also ensured that the data was evenly distributed across the range of values.
PID | Tacticity | CAS# | Chemical name | Calc. FM | Exp. FM | Δ | Orig. reference |
---|---|---|---|---|---|---|---|
Training Set | |||||||
P010001-LDPE | n/a | 9002-88-4 | Polyethene, low density | 0.2455 | 0.188 | 0.0575 | I II |
P010002 | a | 9003-07-0 | Polypropylene | 0.928 | 0.18 | 0.748 | III |
P020001 | a | 9003-53-6 | Polystyrene | 2.3958 | 2.812 | −0.4162 | II |
P040048 | a | 9011-14-7 | Poly(methyl methacrylate) | 3.3301 | 3.1 | 0.2301 | IV |
P040131 | a | 33827-81-5 | Poly(N-dodecylacrylamide) | 0.4207 | 0.1082 | 0.3125 | V |
P040134 | a | 31472-14-7 | Poly(N-octadecylacrylamide) | 0.1943 | 0.2241 | −0.0298 | V |
P044594 | a | 25232-34-2 | Poly[N-(octadec-9-enyl)acrylamide] | 0.0176 | 0.0393 | −0.0217 | V |
P070001 | n/a | 9002-81-7 | Polyformaldehyde | 2.1387 | 2.562 | −0.4233 | VI |
P070337 | n/a | n/a | Poly[oxyethyleneoxycarbonyl-1,4-phenylene(ethylmethylmethylene)-1,4-phenylenecarbonyl] | 2.311 | 2.482 | −0.171 | VII |
P090005 | n/a | 26247-20-1 | Poly(1,4-butylene succinate) | 1.0197 | 0.754 | 0.2657 | VIII |
P090027 | n/a | 25038-59-9 | Poly(ethylene terephthalate) | 2.997 | 2.275 | 0.722 | IX |
P090029 | n/a | 24968-12-5 | Poly(1,4-butylene terephthalate) | 2.2653 | 2.44 | −0.1747 | X |
P090089 | n/a | 25248-42-4 | Poly(epsilon-caprolactone) | 0.3278 | 0.437 | −0.1092 | XI |
P090134 | n/a | 26546-03-2 | Poly(1,3-propylene terephthalate) | 2.6608 | 2.657 | 0.0038 | X XII |
P090176-DL | n/a | 26100-51-6 | Poly( dl -lactic acid) | 3.6415 | 3.585 | 0.0565 | XI |
P090176-L | n/a | 26811-96-1 | Poly( l -lactic acid) | 3.1913 | 3.413 | −0.2217 | XI |
P090559 | n/a | n/a | Poly{[4,4′-(1-methylethane-1,1-diyl)diphenol]-alt-[4,4′-(1-methylpropane-1,1-diyl)dibenzoic acid]} | 2.3332 | 1.862 | 0.4712 | XIII |
P100005 | n/a | 32131-17-2 | Poly[(hexane-1,6-diamine)-alt-(adipic acid)] (Nylon 6,6) | 0.7635 | 1.062 | −0.2985 | XIV |
P100025 | n/a | 24937-16-4 | Poly(dodecano-12-lactam), (Nylon 12, PA-12) | 0.1235 | 0.378 | −0.2545 | XV |
P100088 | n/a | n/a | Poly{(hexane-1,6-diamine)-alt-[4,4′-(1-methylpropane-1,1-diyl)dibenzoic acid]} | 1.7872 | 2.448 | −0.6608 | XIII |
P130124 | n/a | 51518-44-6 | Poly(3,3′-carbonyldiphenylene-3,3′,4,4′-benzophenonetetracarboxydiimide) | 3.8303 | 4.278 | −0.4477 | XVI |
P150011 | n/a | 25037-45-0 | Poly(bisphenol A carbonate) | 2.8182 | 2.179 | 0.6392 | XIII XVII XVIII |
P432568 | n/a | 499204-42-1 | Poly(N-butylnorborn-2-ene-5,6-dicarboximide) | 2.0667 | 2.36 | −0.2933 | XIX |
P432569 | n/a | 308365-06-2 | Poly(N-pentylnorborn-2-ene-5,6-dicarboximide) | 1.629 | 1.53 | 0.099 | XIX |
P432570 | n/a | 308365-07-3 | Poly(N-hexylnorborn-2-ene-5,6-dicarboximide) | 1.3769 | 1.46 | −0.0831 | XIX |
Outliers | |||||||
P010001-HDPE | n/a | 9002-88-4 | Polyethene, high density | 0.2455 | 0.866 | −0.6205 | II |
P010002 | i | 9003-07-0 | Polypropylene | 0.5712 | 1.44 | −0.8688 | II, XX, XXI, XXII |
P020001 | s | 9003-53-6 | Polystyrene | 2.5852 | 4.168 | −1.5828 | XXIII |
P100004 | n/a | 25038-54-4 | Poly(hexano-6-lactam), (Nylon 6) | 0.8463 | 3 | −2.1537 | XXIV |
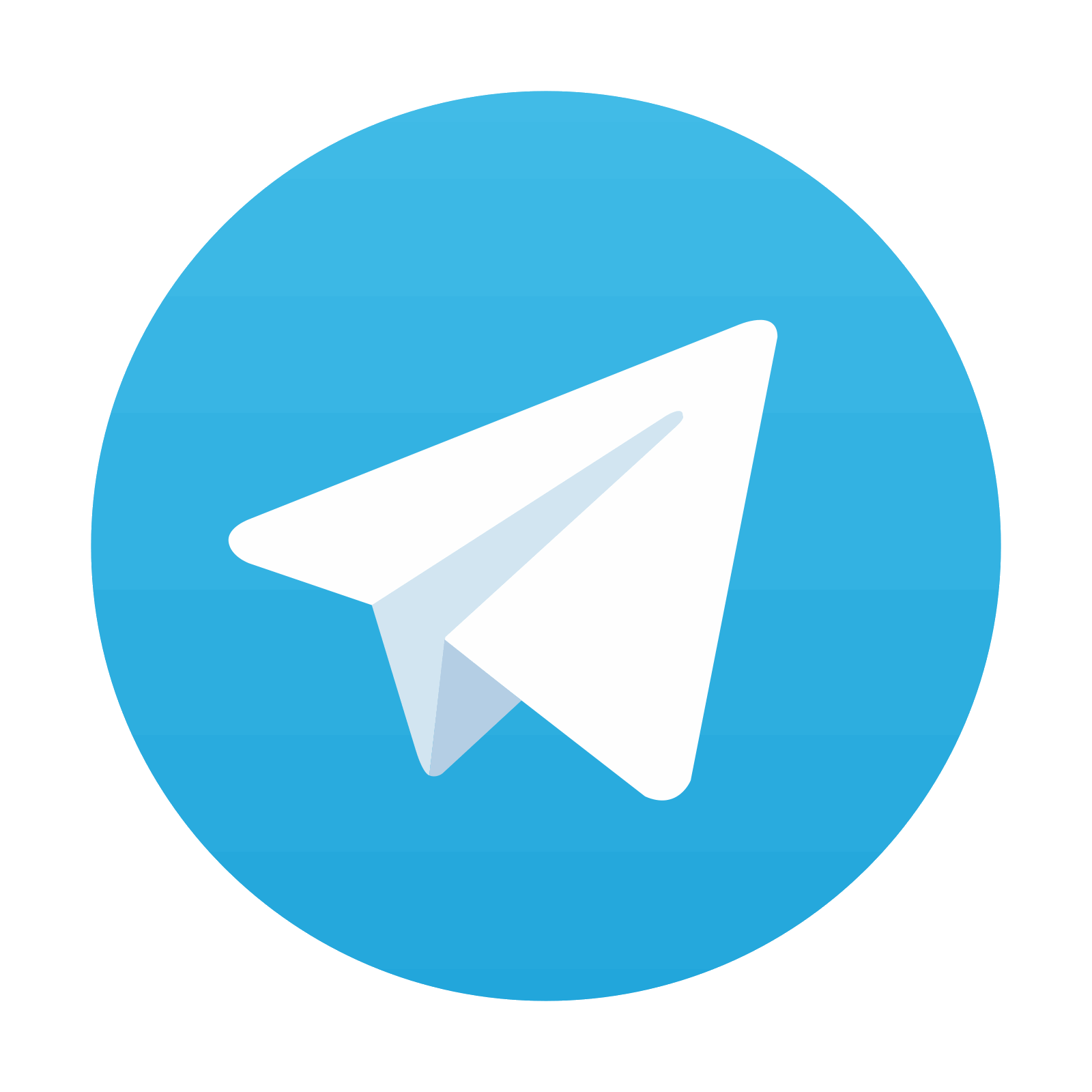
Stay updated, free dental videos. Join our Telegram channel

VIDEdental - Online dental courses
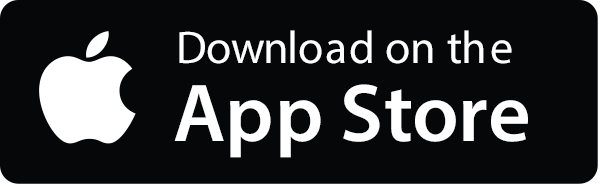
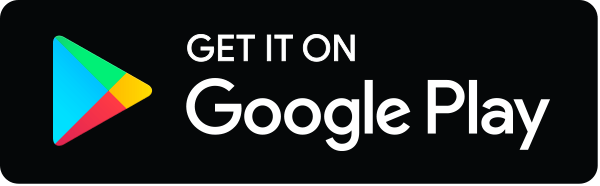
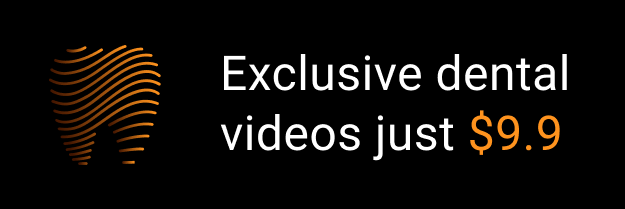