Key Terms
Age hardening Process of hardening certain alloys by controlled heating and cooling, which usually is associated with a phase change.
Annealing The process of controlled heating and cooling that is designed to produce desired properties in a metal. Typically, the annealing process is intended to soften metals, increase their ductility, stabilize shape, and increase machinability.
Antiflux A substance such as graphite that prevents the flow of molten solder on areas coated by the substance.
Base metal A metal that readily oxidizes or corrodes. Important base metals for dental alloys are nickel, cobalt, iron, titanium, and chromium.
Cold welding The metal-joining process by metallic bonding that does not rely on heating to achieve fusion but the pressure applied to the interface between parts to be joined; no liquid phase is produced within the interface joint.
CP Ti Commercially pure titanium, which consists of 99 wt% or higher-purity titanium, with oxygen, carbon, nitrogen, and hydrogen dissolved interstitially.
Dislocation An imperfection in the crystalline arrangement of atoms consisting of an extra partial plane of atoms (edge dislocation), a spiral distortion of normally parallel atom planes (screw dislocation), or a combination of the two types.
Flux Compound applied to metal surfaces that dissolves or prevents the formation of oxides and other undesirable substances that may reduce the quality or strength of a soldered or brazed area.
Grain growth The increase in the mean crystal size of a polycrystalline metal produced by a heat-treatment process.
Grain refinement The process of reducing the crystal (grain) size in a solid metal through the action of specific alloying elements or compounds; the process increases the yield strength of metals.
Lost wax technique Process in which a wax pattern, prepared in the shape of missing tooth structure, is embedded in a casting investment and burned out to produce a mold cavity into which molten metal is cast.
Noble metal Gold and platinum group metals (platinum, palladium, rhodium, ruthenium, iridium, and osmium), which are highly resistant to oxidation and dissolution in inorganic acids.
Precipitation hardening The process of strengthening and hardening a metal by precipitating a phase or constituent from a saturated solid solution.
Recovery A stage of heat treatment that results in the partial restoration of properties of a work-hardened metal without a change in the grain structure.
Recrystallization The process of forming new stress-free crystals in a work-hardened metal through a controlled heat-treatment process.
Soldering Process of building up a localized area of a metal prosthesis with a molten filler metal or joining two or more metal components by heating them to a temperature below their solidus temperature and filling the gap between them using a molten metal with a lower liquidus temperature. If the melting temperature of the solder is greater than 450 °C, the process is called brazing.
Springback The amount of elastic strain that a metal can recover when loaded to and unloaded from its yield strength; an important property of orthodontic wires.
Strain hardening The increase in strength and hardness and decrease in ductility of a metal that is caused by permanent deformation below its recrystallization temperature; also called work hardening or cold working.
Superelasticity The ability of certain nickel-titanium alloys to undergo extensive elastic deformation resulting from a stress-assisted phase transformation, with the reverse transformation occurring on unloading; sometimes called pseudoelasticity.
Welding Process of fusing two or more metal parts through the application of heat, pressure, or both, with and without a filler metal, to produce a localized union across an interface between the workpieces.
Working range The maximum amount of elastic strain that an orthodontic wire can sustain before it permanently deforms.
Wrought alloy A metal that has been permanently deformed to alter the shape of the structure and certain mechanical properties, such as strength, hardness, and ductility.
In his 1907 U.S. Patent 865823, Taggart described a method of making gold inlays using the lost wax technique. The lost wax method of making metal objects involves pouring a molten metal into a mold that has been created with a wax pattern ( Chapter 14, Wax Pattern and Sprue Design ). The process led to making custom precision casts for the restoration of missing tooth structure, such as onlays, crowns, multiple-unit fixed dental prostheses (FDPs), and frameworks for removable partial dentures (RPDs). Since the early 1980s, the numbers of dental alloys, along with new alloy systems, have increased dramatically as a result of the market-price increase of noble metals, the performance of the same function at a lower cost, the need for increasingly specialized physical and mechanical properties, and the awareness of the importance of biological properties.
Objects of any design, intricate or simple, can be produced by using the lost wax technique as long as a wax pattern can be made and invested, and alloys can be melted, or by using alternative processing techniques such as computer-aided design/computer-aided manufacturing (CAD-CAM) milling and three-dimensional (3-D) printing. On the other hand, many ancillary dental materials and instruments are fabricated from initially cast alloys that have been subsequently rolled to form sheets or rods, drawn into wire or tubing, or forged into a finished shape. Rolling, drawing, and forging are major processes of permanently deforming metals.
Whenever a cast pure metal or alloy is permanently deformed to an intended shape in any manner, it is considered a wrought alloy. This permanent deformation alters the microstructure, and the alloy exhibits properties that are different from those it had in the as-cast state. The most significant change is the increase of yield strength with a reduction in ductility. The applications of wrought alloys in dentistry include orthodontic wires, clasps for RPDs, root canal files and reamers, preformed crowns in pediatric dentistry, and surgical instruments. Most metal-based restorations and prostheses are cast and not wrought.
In the construction of dental appliances, there is often a need to join metal parts, cast or wrought, together using high heat. When wrought alloys are involved in such joining, the strength and fracture resistance of the wrought alloy will be compromised if the metal is exposed to the temperature range at which the wrought structure is diminished or lost. Such weakening can occur during the metal-joining process for stainless-steel orthodontic appliances.
The ability to work with dental alloys and associated wrought alloys is dependent on a knowledge of these materials. The goal of this chapter is for the reader to become familiar with various types of casting alloys and associated wrought alloys. We will discuss the desirable properties and the relevant mechanical properties of dental alloys first. For casting alloys, the focus is the alloy classification and clinical applications of the alloys. For wrought alloys, the focus is the process of permanent deformation and its effect on the properties for their specific applications in dentistry. Several types of wrought alloys used in dentistry are described. The description of joining metals is like that for bonding presented in Chapter 6 ; the emphasis is on the principles and procedures of the process.
As previously done in Chapter 2, Metals , the terms metal and alloy are frequently used interchangeably.
Desirable Properties of Dental Alloys
Depending on the primary purpose of the prosthesis, the choice of casting alloy is made by the dentist in collaboration with a qualified dental laboratory technician or technologist. From the standpoint of patient safety and the risk of medico-legal issues, it is highly important to understand the following clinically important requirements and properties of dental casting alloys:
- 1.
Biocompatibility—The alloy must tolerate oral fluids and not release any harmful products into the oral environment that might cause a toxic or allergic reaction. Biocompatibility will be covered in Chapter 17 .
- 2.
Tarnish and Corrosion Resistance— Corrosion is the physical deterioration of a material in the oral environment, and tarnish is a thin film that is adherent to the metal surface ( Chapter 3, Tarnish and Corrosion ). Both phenomena affect the durability and appearance of the prostheses.
- 3.
Thermal Properties—The melting range of the casting alloys must be low enough to form smooth surfaces with the mold wall of the casting investment ( Chapter 14, Investment Materials ). For metal-ceramic prostheses, the alloys must have closely matching thermal expansion coefficients to be compatible with the given porcelains, and they must tolerate high processing temperatures without deforming via a creep process.
- 4.
Strength Requirements—The alloy must have sufficient strength for the intended application. For example, alloys for bridgework require higher strength than alloys for single crowns.
- 5.
Fabrication—The molten alloy should flow freely into the investment mold, without any appreciable interaction with the investment material, and wet the mold to form a surface free of porosity. It should be possible to cut, grind, finish, and polish the alloy to obtain a prosthesis with a satisfactory surface finish.
- 6.
Porcelain Bonding—The alloy must be able to form a thin adherent oxide that enables chemical bonding to ceramic veneering materials.
- 7.
Economic Considerations—The cost of metals used for single-unit prostheses or as frameworks for FDPs or RPDs is a function of the metal density, fluctuations in metal prices, and the cost per unit mass.
Functional Mechanical Properties of Alloys
Mechanical properties are the measured responses of materials in the form of stress and strain under an applied force or distribution of forces. The relevant functional characteristics of casting and wrought alloys are described next.
What are two clinical disadvantages of cast metals that have lower elastic moduli? Why does a long-span bridge require alloys of high elastic modulus?
Elastic Modulus
One characteristic of a material with a high elastic modulus is its rigidity or stiffness. For a dental prosthesis, rigidity is equivalent to the resistance to flexure (bending). When a long-span FDP flexes during occluding of the pontic, the mesiodistal bending moment exerted on the abutment teeth can act as a dislodging force, lifting the mesial and distal aspects of the prosthesis. Furthermore, a flexing bridge can induce lateral forces on the abutment teeth, resulting in the loosening of these teeth. For a metal-ceramic prosthesis, the overlying brittle porcelain will fail catastrophically when the metal substructure flexes beyond the flexural limit of the ceramic. The elastic modulus is also important for the major connectors of an RPD, which must have enough rigidity to prevent flexure during the placement and function of the prosthesis. Resistance to flexure also allows the clasps of an RPD to fit into areas of minimal undercuts and still provide adequate retention.
Why is it that a cast prosthesis that is subjected to tensile stress above the alloy yield strength will not necessarily fracture?
Yield Strength (Proof Stress)
Recall the discussion in Chapter 4, Stress-Strain Properties that yield strength, elastic limit, and proportional limit, by definition, are different properties, but all three terms have been used to reflect the capacity of a cast prosthesis to withstand mechanical stresses without permanent deformation. Ideally, dental alloys should have a high yield strength so that a large amount of stress must be applied before a permanent change in dimensions occurs. For an orthodontic appliance, it relates to the maximum force the wire can deliver. Generally, alloys with tensile yield strengths above 300 MPa function satisfactorily in the mouth.
How does the alloy ductility increase the fracture resistance of a margin of a cast metal crown or a clasp arm on an RPD?
Ductility (Percent Elongation)
Ductility represents the amount of permanent deformation under tensile stress that an alloy can undergo before it fractures. If the force applied is in a compressive mode, the property is called malleability. A reasonable amount of ductility and malleability is essential if a prosthesis requires adjustment to be functional, such as the bending of RPD clasps and the burnishing of crown margins. As discussed earlier, one needs to apply stress greater than the yield strength of the material to cause permanent deformation on a metal surface. Therefore, high ductility allows one to achieve more permanent deformation without fracture but does not indicate if burnishing or adjusting the prosthesis would be easier or more difficult in terms of the stress required.
Why is a harder metal more resistant to wear than a softer metal?
Hardness
Clinically, hardness reflects the resistance of the restoration to scratching and abrasion by the opposing tooth or restoration and the ability to maintain the smoothness of the prosthesis in the oral environment. However, a harder restoration surface can cause excessive wear of softer opposing dentition or restorations. In addition, harder surfaces are more difficult to cut, grind, finish, and polish because higher stress is required for each procedure.
Fatigue Resistance
Fatigue failure can occur when a material is subjected to repeated loading and unloading below its elastic limit. Most fractures of prostheses and restorations develop progressively over many stress cycles of loading and unloading. When the load is above a certain threshold, crack propagation is initiated from flaws within the prosthesis. Eventually, a crack propagates to a critical size, and sudden fracture occurs. Common engineering expressions of fatigue fracture resistance are fatigue strength and endurance limit ( Figure 4.15 ). Fatigue strength (S Nf ) is defined as the stress at which failure occurs after a specific number of fatigue cycles. Endurance limit is the maximum stress that can be maintained without failure over an infinite number of cycles. Some alloys do not have a well-defined endurance limit.
Classification of Dental Casting Alloys
In 1932, the dental materials group at the National Bureau of Standards (now National Institute of Standards and Technology) classified dental gold alloys being used then by Vickers hardness number (VHN): type I (soft, VHN 50 to 90), type II (medium, VHN 90 to 120), type III (hard, VHN 120 to 150), and type IV (extra hard, VHN 150 and above). Since then, the number of alloy compositions and applications has increased vastly. They are now classified according to composition, intended usage, or mechanical properties.
Alloy Classification by Noble Metal Content
In 1984, the American Dental Association (ADA) proposed a simple classification for dental casting alloys based on the content of noble metals. Three categories were described: high noble (HN), noble (N), and predominantly base metal (PB). Subsequently, a fourth group of titanium and titanium alloys was added because of the unique characteristics of titanium. This classification is presented in Table 9-1 . Noble metals comprise a group of seven metals that are resistant to corrosion and tarnish in the mouth. In order of increasing melting temperature, they include gold, palladium, platinum, rhodium, ruthenium, iridium, and osmium. These noble metals and silver are sometimes called precious metals, referring to their high economic value, but the term precious is not synonymous with noble. Silver is reactive in the oral cavity and is not a noble metal.
Alloy Type | Total Noble-Metal Content |
High noble (HN) | Must contain ≥40% Au and ≥60% by weight of noble-metal elements * |
Noble (N) | Must contain ≥25% by weight of noble-metal elements |
Predominantly base metal (PB) | Contains <25% by weight of noble-metal elements |
Titanium and titanium alloys | Titanium ≥85% by weight |
* Noble-metal elements include Au, Pd, Pt, Rh, Ru, Ir, and Os.
Based on this classification, the IdentAlloy program was established by manufacturers to provide documentation of certified alloys. Under this program, each alloy has a certificate ( Figure 9-1 ) that lists its manufacturer, alloy name, composition, and ADA classification. Some insurance companies use it as well to determine the cost of crown and bridge treatment. Keep in mind that this system certifies only the composition of alloys.

Alloy Classification by Mechanical Properties
The historic ADA Specification No. 5, which originally had composition ranges for the component elements in the four types of gold casting alloys, evolved to stipulating mechanical property requirements for yield strength, percentage elongation, and Vickers hardness. The relevant current standard is International Organization for Standardization (ISO) 22674:2016, which classifies metallic materials for fixed and removable restorations and appliances into six types according to yield strength and elongation, regardless of noble metal contents ( Table 9-2 ).
Type | Yield Strength (MPa) | Elongation (%) | Examples of Applications |
---|---|---|---|
0 * | — | — | Single-tooth fixed restorations—for example, small veneered one-surface inlays, veneered crowns |
1 | 80 | 18 | Single-tooth fixed restorations, veneered or nonveneered one-surface inlays, veneered crowns |
2 | 180 | 10 | Single-tooth fixed restorations—for example, crowns or inlays without restriction on the number of surfaces |
3 | 270 | 5 | Multiple-unit fixed restorations—for example, bridges |
4 | 360 | 2 | Appliances with thin cross sections that are subjected to very high forces—for example, RPDs, clasps, thin veneered crowns, wide-span bridges or bridges with small cross sections, bars, attachments, implant-retained superstructures |
5 | 500 | 2 | Thin RPDs, parts with tin cross sections, clasps |
* Metallic materials for metal-ceramic crowns produced by electroforming or sintering belong to type 0.
Alloy Classification by Principal Elements
Alloys may be classified based on the principal or most abundant element (e.g., a palladium-based alloy), or they may be named based on the two or three most important elements (e.g., Pd-Ag, Co-Cr, or Ni-Cr-Be alloys). The components are listed in decreasing order of weight percent. An exception to this rule is the identification of elements that substantially affect the properties of the alloy. For example, Ni-Cr-Mo-Be alloys are often designated as Ni-Cr-Be alloys because of the contributions of beryllium to the control of castability and surface oxidation at high temperatures and the relative health-hazard potential of beryllium compared with other metals. Castability refers to the ease of making quality castings. Excellent websites to view the wide range of dental alloy compositions include Argen at https://argen.com/ .
Alloy Classification by Dental Applications
There are three categories of dental casting alloys designated by application: all-metal fixed prostheses, metal-ceramic prostheses, and RPDs. Each type of alloy classified by noble metal content ( Table 9-1 ) is available in all three categories. One may use high-noble and noble alloys intended for metal-ceramic prostheses to fabricate all-metal prostheses. However, alloys for all-metal restorations, which can deform or melt during porcelain-firing, should not be used to make metal-ceramic restorations.
Base-metal and titanium alloys, on the other hand, are often marketed for both all-metal and metal-ceramic prostheses because of their oxide formation at room temperature. In this chapter, dental alloys are discussed by their applications.
Alloys for All-Metal Prostheses
These alloys are discussed in three main categories: noble (includes high-noble), predominantly base-metal, and titanium and titanium alloys.
High-Noble and Noble Alloys
The compositions of selected high-noble and noble alloys are given in Table 9-3 . Their physical and mechanical properties are shown in Table 9-4 . Gold and palladium are the two major elements that make up the nobility of noble and high-noble alloys; these alloys are discussed in two categories: Au-based alloys and Pd-based alloys.
ELEMENTAL COMPOSITION (PERCENT BY WEIGHT) | ||||||||
---|---|---|---|---|---|---|---|---|
Alloy Type | Color | Au | Pd | Ag | Cu | Pt | In | Balance * |
1 | Yellow | 83 | 0.5 | 10 | 6 | — | — | Zn; Ir |
2 | Yellow | 82 | — | — | — | 18 | — | Ir |
Yellow | 77 | 1 | 13 | 8.5 | — | — | Zn; Ir | |
3 | Yellow | 74.5 | 3.5 | 11 | 10.5 | — | — | Zn; Ir |
Yellow | 50 | 4 | 35 | 10 | — | — | Zn; Ir | |
Yellow | 2 | 34.9 | 30 | — | — | 30 | Zn:3; Ir | |
White | — | 25 | 71.5 | — | — | 2.5 | Zn; Ru | |
4 | Yellow | 60 | 4 | 20.5 | 15 | — | — | Zn; Ir |
Yellow | 50 | 3 | 26 | 11.5 | — | 3 | Zn:1; Ir | |
Pale yellow | 20 | 20 | 36 | — | — | 18 | Zn:6; Ir | |
White | 4 | 30.3 | 45.7 | 18.5 | — | — | Zn:1.5; Ir |
Alloy Type | ADA Classification | Density (g/cm 3 ) | Yield Strength (MPa; soft/hard) | Hardness (VHN; soft/hard) | Elastic Modulus (GPa) | Elongation (%; soft/hard) |
---|---|---|---|---|---|---|
1 | High noble | 16.6 | 126 | 85 | 70 | 51 |
2 | High noble | 19.2 | 146 | 95 | 50 | 23 |
High noble | 15.4 | 221 | 120 | 109 | 54 | |
3 | High noble | 15.5 | 207/276 | 121/182 | 76 | 39/19 |
Noble | 13.2 | 309/648 | 138/225 | 104 | 28/15 | |
Noble | 10.5 | 297 | 180 | 51 | 5–6 | |
Noble | 10.5 | 248/310 | 145/155 | 55 | 10/8 | |
4 | High noble | 14.5 | 350/607 | 165/235 | 88 | 35/4 |
Noble | 13.2 | 428/683 | 180/255 | 86 | 28/16 | |
Noble | 11.3 | 420/530 | 165/220 | 103 | 10/2 | |
Noble | 10.6 | 460/700 | 195/265 | 116 | 10/3 |
Gold-Based Alloys
Referring to Table 9-2 , type 1 alloys are designed for inlays that are not subjected to substantial mastication forces. Type 2 alloys having greater mechanical properties than type 1 are used for one-surface inlays. Type 3 alloys are used for constructing crowns, bridge abutments, and inlays, which are subjected to great stress during mastication. Type 4 alloys are used in high-stress areas such as bridges and RPD frameworks (although the gold-based RPD is now rare). The alloys for bridge and RPD framework constructions must be sufficiently rigid to resist flexure, possess high yield strength to prevent permanent distortion, and be ductile enough for adjustment if the clasp of a framework has been distorted or needs adjustment.
Various elements have been incorporated into gold alloys to produce alloys with suitable properties. For example, platinum and palladium increase the hardness and elastic modulus of gold and raise the melting temperature of the alloy. A higher melting temperature is beneficial when components are to be joined by soldering. Copper in a sufficient quantity relative to the gold content renders the alloy heat-treatable ( Chapter 2, Gold-Copper System ) but can alter the color of the alloy. Silver forms solid solutions with gold and palladium and is effective in neutralizing the reddish color of copper in gold-copper alloys. Palladium has a good range of solubility with gold, silver, and copper, resulting in good mechanical properties and improvement of tarnish and corrosion resistance. Zinc acts as an oxygen scavenger during the melting and casting of noble and high-noble alloys.
What effects can the cooling rate of a heat-treated type 3 gold-copper alloy have on its hardness and ductility?
What happens within gold-copper alloys that are subjected to a prescribed heating–cooling cycle?
The underlying principles for heat treatment of these alloys have been discussed in Chapter 2, Gold-Copper System . Briefly, the casting is placed in a furnace at 700 °C for 10 minutes, and then quenched in water to retain a disordered solid-solution phase with reduced yield strength and hardness but increased ductility. The process is called a solution heat treatment. The same casting is placed in a furnace with the temperature set between 200 and 450 °C for 15 to 30 minutes before quenching in water. The treatment produces regions of ordered solid-solution phase with increased yield strength and hardness but a reduction of elongation ( Table 9-4 ). The process is called age hardening.
The softening heat treatment is indicated for prostheses that are ground or reshaped (permanent deformation) to a different form, either in or out of the mouth, to restore their ductility. Because the proportional limit is increased during age hardening, a considerable increase in the modulus of resilience can be expected. The hardening heat treatment is indicated for metallic RPDs, saddles, FDPs, and other similar structures where rigidity of the prosthesis is needed. For small structures, such as inlays, a hardening treatment is not usually required.
What characteristics of palladium make it a natural choice of element to replace gold for dental alloys?
Silver-Palladium Alloys
Silver-palladium alloys are often white and predominantly silver in composition and must contain at least 25% palladium to provide tarnish and corrosion resistance of the alloy. They may also contain copper and a small amount of gold to increase the ductility and improve the castability of the alloy for dental applications. Ag-Pd alloys can produce acceptable castings when close attention is paid to precise control of the casting and mold temperatures. Casting temperatures are in the range of those for yellow gold alloys. The copper-free Ag-Pd alloys may have physical properties similar to those of a type 3 gold alloy. With 15% or more copper, the alloy may have properties more like those of a type 4 gold alloy.
Indium and palladium at a 1:1 atomic ratio form a gold-color intermetallic compound. When the content of indium in a Pd-Ag alloy is 18% to 30% by weight, the alloy achieves a gold-like color. The colored phase of the Pd-In binary alloy system is hard and brittle and is not a strengthener. Iridium or ruthenium is added in small quantities to high-noble and noble alloy compositions for grain refinement because smaller grains improve the yield strength ( Chapter 2, Grain Refinement and Grain Size ).
What is the role of chromium in predominantly base-metal alloys? How does beryllium improve the properties of nickel-based alloys?
Predominantly Base-Metal Alloys
Currently, there are two main groups of base-metal dental alloys: nickel-chromium (Ni-Cr) and cobalt-chromium (Co-Cr). The Ni-Cr alloys can be further divided into those with and without beryllium, which improves castability and promotes the formation of a stable metal oxide for porcelain bonding. The majority of Ni-Cr alloys are used for small castings such as crowns and FDPs, and Co-Cr alloys are primarily used for casting RPDs for which a high elastic modulus and yield strength are needed. Table 9-5 lists the compositions and properties of selected base-metal alloys for all-metal and metal-ceramic applications.
COMPOSITION (PERCENT WT) | MECHANICAL PROPERTIES | ||||||||
---|---|---|---|---|---|---|---|---|---|
Alloy (Supplier) | Ni | Co | Cr | Mo | Balance * | Yield Strength (MPa) | Hardness (VHN) | Elastic Modulus (GPa) | Elongation (%) |
IPS d.Sign 15 (Ivoclar) | 58.7 | 1 | 25 | 12.1 | Fe:1.9; Si:1.7; Ce | 340 | 230 | 200 | 13 |
Rexalloy (Pentron Alloys/Argen) | 67 | — | 14 | 8 | Ga:8; Al; Fe; Si; Mn; Zr; Cu | 300 | 177 | 191 | 27 |
Heraenium S (Heraeus Kulzer) | 62.9 | — | 23 | 10 | Si:2; Fe:15.5; Ce:0.5 | 310 | 220 | 224 | 29 |
NPX-III (CMP Industries) | 76.5 | x † | 14 | 4.5 | Al:2.5; Be:1.6; Ti | 784 | 350 | 200 | 9 |
Argeloy Bond (Argen) | 77 | — | 14 | 4.7 | Mn; Fe; Si; C | 630 | 370 | 207 | 10 |
Norex (Pentron Alloys/Argen) | — | 55 | 25 | — | W:10; Ru:5; Al, Nb, Y, Zr | 621 | 350 | 204 | 7 |
Heraenium P (Heraeus Kulzer) | — | 59 | 25 | 4 | W:10; Si:1; Mn:0.8; N | 650 | 330 | 200 | 8 |
Jelbond Supreme (Jelenko Alloys/Argen) | — | 61 | 27 | 6 | W:5; Mn; Si; Fe; C | 475 | 365 | 223 | 8 |
Chromium, a critical element of base-metal alloys, oxidizes rapidly on the surface of the alloy to form a thin layer of chromium oxide, which prevents the diffusion of oxygen into the underlying metal and the corrosion process. Chromium in the bulk alloy also provides strengthening by solid-solution hardening. Molybdenum increases corrosion resistance and strength and decreases the thermal expansion coefficient of base-metal alloys. Higher thermal expansion is beneficial for porcelain bonding and minimizes the risk of porcelain cracking or fracture. Some minor elements are incorporated to improve manipulation and mechanical properties. For example, beryllium lowers the fusion temperature of the alloys, which improves castability, and refines grain size, which increases strength. Manganese and silicon also improve the castability of the alloys. Carbon forms carbides with other elements in the alloy, increasing the hardness and yield strength with a reduction in ductility. The aluminum in Ni-Cr alloys forms a nickel-aluminum compound, Ni 3 Al, which increases both the yield strength and the tensile strength. Nitrogen, which is incorporated in the alloy during casting in ambient air, also improves the overall qualities of the casting.
The manipulation and mechanical properties of base-metal alloys are described in more detail in the following section on alloys for metal-ceramic prostheses and in the subsequent section on RPDs because base metals are used to a lesser extent for all-metal prostheses.
Titanium and Titanium Alloys
Titanium has a high melting point (1668 °C) and a high rate of oxidation above 900 °C. It requires a special casting machine with high-temperature melting capability in a nonoxidizing chamber. For example, an argon arc with a nonconsumable tungsten electrode or high-frequency induction is used for melting titanium alloys in crucibles made of copper, magnesia, or carbon and in an argon or helium atmosphere. A casting investment consisting of oxides, such as MgO, ZrO 2 , or Y 2 O 3 , which are more stable than titanium oxide, is used to ensure acceptable castability. Centrifugal force, casting-pressure difference, and gas pressure have been used to force the molten-metal flow into the mold. Titanium can react with the investment material during casting and forms a very hard surface layer called α-case, with a thickness up to 150 μm. The VHN of cast commercially pure titanium increases from a bulk value of nearly 200 to approximately 650 at a depth of 25 μm below the surface. Special instruments are required in the dental laboratory for finishing and adjusting titanium castings.
Titanium derives its corrosion protection from a thin passivating oxide film (about 10 nm thick), which forms spontaneously with the surrounding oxygen. Titanium is considered the most biocompatible metal used for dental restorations and prostheses.
Commercially Pure Titanium
According to the American Society for Testing and Materials (ASTM) Standard F67, there are four unalloyed grades of commercially pure titanium (CP Ti) based on the concentration of impurities ( Table 9-6 ). The elastic modulus of CP Ti is comparable to that of tooth enamel and noble alloys, but it is lower than that of other base-metal alloys ( Table 9-5 ). CP Ti is often selected for its excellent corrosion resistance, especially in applications for which high strength is not required. The major strengthening element is oxygen. Increasing the oxygen content of CP Ti increases not only the flexural strength but also the fatigue strength.
PHYSICAL PROPERTIES (MINIMUM) | IMPURITY LIMITS (max), PERCENT BY WEIGHT | ||||||||
---|---|---|---|---|---|---|---|---|---|
Designation | Tensile Strength (MPa) | Yield Strength (MPa) | Hardness (VHN) | Elongation (%) | N | C | H | Fe | O |
Grade 1 | 240 | 170 | 126 | 24 | 0.03 | 0.08 | 0.015 | 0.20 | 0.18 |
Grade 2 | 340 | 280 | 178 | 20 | 0.03 | 0.08 | 0.015 | 0.30 | 0.25 |
Grade 3 | 450 | 380 | 221 | 18 | 0.05 | 0.08 | 0.015 | 0.30 | 0.35 |
Grade 4 | 550 | 480 | 263 | 15 | 0.05 | 0.08 | 0.015 | 0.50 | 0.40 |
Why is a Ti-Al-V alloy with high strength not always preferred to the commercially pure titanium metal for dental prostheses? How do alloying elements affect the properties of titanium dental alloys?
Titanium Alloys
CP Ti undergoes an allotropic (polymorphic) transformation from a hexagonal close-packed crystal structure (α phase) at 882 °C to a more ductile body-centered crystal structure (β phase). By incorporating a combination of α- and β-phase stabilizers, four possible types of titanium alloys can be produced: α, near-α, α-β, and β. Alpha-phase stabilizers, such as aluminum, carbon, nitrogen, and gallium, raise the temperature for transforming α to β phase on heating. Beta-phase stabilizers, such as molybdenum, cobalt, nickel, niobium, copper, palladium, tantalum, and vanadium, lower the temperature for transforming β to α phase on cooling. Alpha alloys will form no β phase on cooling. Near-alpha alloys will form a limited amount of β phase on cooling. Alpha-beta alloys are metastable, which makes them heat-treatable and will contain both phases at room temperature. Thermal treatment dictates the relative amount of α and β phases and mechanical properties. Beta alloys contain sufficient beta stabilizers to retain the β phase on cooling; they can be solution-treated and aged to improve strength.
The most widely used titanium alloy in dentistry and for general engineering applications is Ti-6Al-4V, which is an α-β alloy. Although this alloy has greater strength than CP Ti, it is not as attractive for dentistry and biomedical applications because of some concerns about health hazards from the slow release of aluminum and vanadium. Vanadium in high doses is highly toxic, and aluminum has been reported to cause potential neurological disorders. Niobium has not been associated with any known toxic or adverse reactions in the body and belongs to the same group as vanadium in the periodic table. Replacing vanadium in Ti-6Al-4V with the same atomic percentage of niobium yields Ti-6Al-7Nb. Both alloys are considered to be acceptable for biomedical applications. The mechanical properties of the two alloys are similar ( Table 9-7 ), and their corrosion resistance is similar to that of CP Ti. Their elastic moduli are similar to those of type 4 alloys, and yield strengths range from those of type 2 to type 5 metallic materials, as described in ISO 22674 ( Table 9-2 ).
Alloy | Elastic Modulus (GPa) | Yield Strength (MPa) | Hardness (VHN) | Elongation (%) |
---|---|---|---|---|
Ti-6Al-4V | 117 | 860 | 320 | 10–15 |
Ti-6Al-7Nb | 105 | 795 | 330 | 10 |
Why does the manipulation of titanium alloys differ so much from that for Co-Cr and Ni-Cr alloys?
Manipulation of Titanium Alloys
The surface of titanium castings after divestment must be ground and polished to remove surface porosity and the α case for optimal functionality of the final prosthesis. The machinability of titanium and its alloys is generally considered poor because of several inherent properties of titanium, such as high chemical reactivity, relatively low thermal conductivity, high strength at high temperature, and low modulus of elasticity.
To eliminate the effect of oxygen during the fabrication of titanium prostheses, CAD-CAM has been used to produce titanium-based fixed prosthesis frameworks.
How do alloys for all-metal prostheses differ from those required for metal-ceramic prostheses?
Alloys for Metal-Ceramic Prostheses
The chief objections to the use of dental porcelain as a restorative material are its low tensile strength and shear strength ( Chapter 10, Mechanical Properties ). A method of minimizing this disadvantage is to bond the porcelain directly to a cast alloy substructure made to fit the prepared tooth. The process requires building on the metal substrate a tooth form with ceramic powder held together by a binder and subjecting the entire assembly to a heating process called sintering. Sintering densifies the packed ceramic particles to a solid firmly bonded to the metal substrate. This system is referred to as the porcelain-fused-to-metal (PFM) design. The preferred descriptive term for this composite system is metal-ceramic, and the metal is called metal-ceramic alloy. In this section, the requirements of metals, some representative alloy systems, and issues associated with these alloys are discussed.
Requirements of Alloys for Metal-Ceramic Applications
The alloys discussed in this section share at least three common features: (1) the potential to bond to dental porcelain, (2) solidus temperature sufficiently high to resist softening during sintering, and (3) coefficients of thermal contraction compatible with those of dental porcelain.
Porcelain Bonding to Metals
Porcelain bonding to metal substrate requires adherent oxides on the surface. The addition of a small quantity of an appropriate base metal to noble and high-noble alloys promotes oxide formation on the surface. For base-metal alloys, some oxides may be poorly adherent to the metal substructure, which can result in porcelain delaminating from the metal substrate. However, the bond strengths for porcelain bonded to base-metal alloys as determined in vitro have not generally been shown to be superior or inferior to those of noble-metal alloys. Furthermore, clinical studies have not demonstrated a significant difference in the failure incidence between metal-ceramic restorations made from base-metal alloys and those fabricated from high-noble or noble-metal alloys. To produce the optimal metal oxide characteristics, the manufacturer’s instructions must be followed precisely.
Solidus Temperature
When an alloy is heated close to its solidus temperature, it becomes susceptible to deformation under its own mass, which is known as creep. The degree of creep increases with the size of the prosthesis and the number of firings required for the porcelain. In the older literature, this creep process is referred to as the sag of the ceramic alloy ( Figure 9-2 ). All metal-ceramic alloys should have a solidus temperature that is substantially higher than the sintering temperature of the porcelain to minimize creep deformation.

How can differences in thermal contraction between a metal and its veneering ceramic affect the resistance to cracking or fracture of the veneer?
Why must a metal for metal-ceramic prostheses have its thermal contraction coefficient slightly higher than that of its veneering ceramic?
Thermal Compatibility of Metal-Ceramic Systems
The thermal expansion and contraction values of base-metal alloys are generally similar to those of noble-metal alloys. When a metal-ceramic prosthesis is cooled from the porcelain sintering temperature, the metal and its veneering ceramic contract at different rates. Meanwhile, the chemical bond between the metal and the porcelain prevents the two components from separating; this condition forces the two components to adjust their respective dimensions during the cooling cycle. Thus the component that contracts more will be stretched by the adjacent component, which contracts less; together, the material that contracts less will be compressed by the other component. Such changes in dimension result in stresses that act on each of the two components. The instantaneous stress at a given temperature during the cooling cycle is termed transient stress, and the stress distribution, which exists at room temperature, is called the residual stress. When the prosthesis is cooled, the tensile stress that develops in the porcelain is of concern. If the transient tensile stresses that develop within porcelain during cooling are insufficient to cause immediate cracking of the porcelain or delayed cracking after cooling to room temperature, the combination of a metal-porcelain system is considered thermally compatible. In fact, a slight thermal contraction mismatch, with a coefficient of thermal contraction of about 0.5 × 10 –6 /°C higher for the metal, is preferred because the mismatch induces residual compressive stress in the porcelain along the bonded interface, which is protective in nature. This mechanism will be discussed in Chapter 10, Coefficient of Thermal Expansion Mismatch .
What are the benefits and drawbacks of the different types of alloys used for metal-ceramic prostheses?
High-Noble and Noble Alloys
Table 9-8 shows some typical high-noble and noble metal–ceramic alloys. The physical and mechanical properties of representative alloys are given in Table 9-9 .
PRINCIPAL ELEMENTS (WEIGHT PERCENT) | ||||||||
---|---|---|---|---|---|---|---|---|
Alloy Type | Typical Products (Supplier) | Au | Pt | Pd | Ag | Cu | Ga | Balance * |
Au-Pd-Pt/HNAu-Pt-Pd/HNAu-Pt-Ag/HN | SMG-3 (Dentsply Ceramco) † | 81 | 6 | 11 | —* | — | — | Re; Sn; Fe |
Argedent Y86 (Argen) † | 86 | 10 | 2 | — | — | — | In:2 | |
Degunorm (Dentsply Ceramco) † | 74 | 9 | — | 9 | 4.4 | — | Zn; In; Ir | |
Au-Pd-Ag/HN | Argedent 75 (Argen) † | 75 | — | 12 | 10 | — | — | In:1; Sn:1; Ir |
Aspire (Dentsply Ceramco) | 52 | — | 26 | 17 | — | — | Zn; In; Sn; Re | |
Herabond (Heraeus-Kulzer) | 52 | — | 27 | 18 | 0.2 | — | Sn:2.7; In; Ir; Re | |
Au-Pd/HN | Olympia (Argen) | 52 | — | 38 | — | — | 1.5 | In:8.5; Ru |
Lodestar (Ivoclar Vivadent) | 52 | — | 39 | — | — | 1.5 | In:8.5; Re; Ru | |
Argedent 65SF (Argen) | 65 | — | 26 | — | — | — | In:8.7 | |
Pd-Au/N | Argedent 35SF (Argen) | 35 | — | 57 | — | — | 4.8 | Sn:3; Zn; Ru |
Pd-Au-Ag/N | Argelite 52+ (Argen) | 15 | — | 52.2 | 21.5 | — | 1 | In:6; Sn:4 |
Argelite 75+6 (Argen) | 6 | — | 75 | 6.5 | — | — | In:6; Ga:6 | |
Pd-Ag/N | Albabond A (Heraeus-Kulzer) | — | — | 57 | 33 | — | — | Sn:6.8; In:3.4; Zn; Ir; Ru |
W-1 (Ivoclar Vivadent) | — | — | 53 | 38 | — | — | Sn:8.5; In; Ru; Li | |
Pd-Cu-Ga/N | Spartan Plus (Ivoclar Vivadent) | 2 | — | 78.8 | — | 10 | 9 | Ir; Ge; Li |
Pd-Ga/N | Argebond 80 (Argen) | — | — | 80 | 5 | — | 6.3 | In:6.5; Sn; Zn:1 |
Argelite 85 (Argen) | 2 | — | 85 | 1.2 | — | 10 | In:1.2; Ru |
Alloy Type | Product Name | Density (g/cm 3 ) | Yield Strength (MPa; Soft/Hard) | Hardness (VHN; Soft/Hard) | Elastic Modulus (GPa) | Elongation (Soft/Hard) (%) |
---|---|---|---|---|---|---|
Au-Pt-Ag | Argedent Y86 | 18.4 | 405/469 | 160/195 | 76 | 12/9 |
Au-Pd-Ag | Herabond | 14.5 | 520/600 | 220/260 | 134 | 12/8 |
Au-Pd | Olympia | 14.4 | 550 | 250 | 124 | 30 |
Pd-Au | Argedent 35SF | 13.9 | 510/572 | 245/245 | 124 | 25/20 |
Pd-Au-Ag | Argelite 75+6 | 11.4 | 565/600 | 250/265 | 111 | 32/30 |
Pd-Ag | Albabond A | 11.4 | 460/540 | 205/235 | 122 | 26/18 |
Pd-Cu-Ga | Spartan Plus | 11.0 | 740 | 310 | 140 | 10 |
Pd-Ga | Argebond 80 | 11.2 | 585/630 | 230/245 | 130 | 35/28 |
Gold-Platinum-Palladium Alloys
Gold-platinum-palladium alloys produced the first successful metal-ceramic restorations. Platinum raises the melting temperature. Rhenium (Re) is a grain refiner that increases hardness. Iron forms an oxide on the surface for porcelain bonding and increases the proportional limit and strength of the alloy by an FePt 3 precipitate. These alloys have adequate elastic modulus, strength, hardness, and elongation but are low in sag resistance. They are mostly used for making crowns and three-unit FDPs. Their use has decreased over time because more economical alloys have been developed with substantially better mechanical properties.
Gold-Palladium-Silver Alloys
Gold-palladium-silver alloys are economical alternatives to the Au-Pt-Pd or Au-Pd-Pt alloys. Their excellent tarnish and corrosion resistance and relative freedom from technique sensitivity have contributed to their long-term success. The higher palladium concentration above those of the Au-Pt-Pd alloys raises the melting ranges and improves resistance to creep deformation at elevated temperatures.
Gold-Palladium Alloys
These silver-free alloys are white in color and have a lower thermal contraction coefficient than that of either the Au-Pd-Ag or Pd-Ag alloys. When used with compatible low-expansion or low-contraction porcelains, Au-Pd alloys are considered nearly ideal compared with other noble-metal alloys because these alloys contain no silver, and their surface oxide is virtually indiscernible. Thus the esthetic quality of metal-ceramic prostheses made with Au-Pd alloys is comparable to that obtained with Au-Pt-Pd alloys. The sag resistance of these alloys is somewhat better than that of Au-Pt-Pd alloys.
Palladium-Gold Alloys
The physical properties of palladium-gold alloys are generally similar to those of the Au-Pd alloys, and potential thermal contraction concerns for these alloys seem to have been resolved by changes in the porcelain composition. Few data are available on their laboratory and clinical performance.
Palladium-Gold-Silver Alloys
Palladium-gold-silver alloys are similar to the Au-Pd-Ag alloys in their potential for porcelain discoloration. They have a range of thermal contraction coefficients, which increase with an increase in silver content.
Palladium-Silver Alloys
This alloy type was the first gold-free noble alloy for metal-ceramic restorations. The replacement of gold by palladium raises the melting range but lowers the thermal expansion coefficient of the alloy. Silver, on the other hand, lowers the melting range but increases the thermal expansion coefficient. A proper balance of the two elements maintains a reasonably low casting temperature and a compatible thermal expansion coefficient. The thermal compatibility of these alloys is generally good except with certain low-expansion porcelains. The addition of tin and indium promotes oxide formation for porcelain bonding and yields precipitates for improving mechanical properties.
Adherence to porcelain is considered acceptable for most of the Pd-Ag alloys. However, Mackert et al. reported that one of these alloy products formed an internal oxide rather than the preferred external oxide. Instead, Pd-Ag nodules developed on the surface ( Figure 9-3 ) facilitate the retention of porcelain mechanically. This condition has not apparently produced clinical bond failures to warrant concern, and other Pd-Ag ceramic alloys have not been studied to determine whether they have similar oxidation behavior.
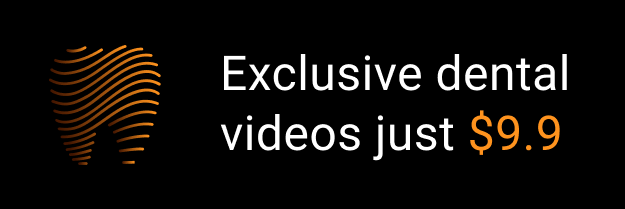