Abstract
Objective
Several features necessary for polymer composite materials in practical applications such as dental restorative materials were investigated in photo-curable CuAAC (copper(I)-catalyzed azide-alkyne cycloaddition) thermosetting resin-based composites with varying filler loadings and compared to a conventional BisGMA/TEGDMA based composite.
Methods
Tri-functional alkyne and di-functional azide monomers were synthesized for CuAAC resins and incorporated with alkyne-functionalized glass microfillers for CuAAC composites. Polymerization kinetics, in situ temperature change, and shrinkage stress were monitored simultaneously with a tensometer coupled with FTIR spectroscopy and a data-logging thermocouple. The glass transition temperature was analyzed by dynamic mechanical analysis. Flexural modulus/strength and flexural toughness were characterized in three-point bending on a universal testing machine.
Results
The photo-CuAAC polymerization of composites containing between 0 and 60 wt% microfiller achieved ∼99% conversion with a dramatic reduction in the maximum heat of reaction (∼20 °C decrease) for the 60 wt% filled CuAAC composites as compared with the unfilled CuAAC resin. CuAAC composites with 60 wt% microfiller generated more than twice lower shrinkage stress of 0.43 ± 0.01 MPa, equivalent flexural modulus of 6.1 ± 0.7 GPa, equivalent flexural strength of 107 ± 9 MPa, and more than 10 times higher energy absorption of 10 ± 1 MJ m −3 when strained to 11% relative to BisGMA-based composites at equivalent filler loadings.
Significance
Mechanically robust and highly tough, photo-polymerized CuAAC composites with reduced shrinkage stress and a modest reaction exotherm were generated and resulted in essentially complete conversion.
1
Introduction
Conventional resin-based dental restorative composites are formulated predominantly from inorganic fillers dispersed in matrices comprised of BisGMA or other related dimethacrylate-based monomer mixtures. These composites are esthetically appealing, mechanically stiff, and biocompatible thermosetting materials with rapid photo-curing kinetics upon incident visible light activation . Typically, a high volume fraction of inorganic silica/glass fillers is used in combination with the resin in dental composites, for instance, 30–65 v/v% or 40–80 wt/wt% of 0.4–1.0 μm fillers/resins . The roles of the filler involve enhanced mechanical performance including properties such as hardness, strength, and wear resistance, along with improved biocompatibility and moisture resistance as well as reduced thermal expansion coefficient and polymerization-induced volumetric shrinkage that leads to significant stress . However, extensive filler loading in resin-based composites can hinder photopolymerization kinetics due to light scattering and also influence handling properties due to the substantial increase in initial viscosity of the uncured composite paste as compared to the unfilled resin . Therefore, the influence of filler types, shapes, sizes, concentrations, and functionalization on kinetics, mechanics, rheology, biocompatibility, and handling properties of resin-based composites has been investigated widely in the search for a more durable and practical dental restorative composite material .
Different classifications of inorganic fillers include metal oxides ( e.g. : silica, aluminum oxide, titanium dioxide), alkaline silicate glass, bioactive or biomimetic fillers ( e.g. : hydroxyapatite), and organic-inorganic hybrids . Silicon dioxides are often utilized in resin-based dental composites due to their mechanical performance along with reduced water-sorption and solubility . Chemical functionalization of the filler that leads to covalent interactions between the filler particles and the polymer matrix after polymerization improves mechanical performance and hydrophobicity of composites as well as handling properties of the uncured pastes . Silane derivatives with reactive functional groups, e.g., methacrylates, are used as coupling agents to chemically modify the surface of inorganic particles, enhance particle dispersion, and promote interfacial bonding between the resin and filler via copolymerization . In addition to bulk silica particles, mesoporous silica nanofillers have also been utilized where their porous structure facilitates mechanical interlocking with the polymerizable resin phase . In contrast to micro-sized fillers (0.1–5 μm) with maximum particle loadings of 70 vol% or 85 wt%, nano-sized fillers (5–100 nm) are more restricted with respect to the maximum loading levels in photo-curable dental composites due to particle aggregation, viscosity increase, and light scattering associated with the significant increase in specific surface area of the particles . As a consequence, nano-filled composites typically have reduced mechanical performance while enabling other positive attributes related to reduced opacity and improved polishability, hardness and wear resistance . Alternatively, hybrid fillers consisting of both micro- and nano-sized particles are often employed in resin-based composites with enhanced packing fraction and surface smoothness .
Despite significant advances in filler development, failure of practical resin-based dental composites have been associated with high polymerization shrinkage stress , brittleness , monomer toxicity, and extractables as well as the formation of biofilms . These issues, which shorten the service lifetime of composite restoratives, arise in part due to the nature of chain-growth polymerization associated with the conventional curing of methacrylate-based composites . The free-radical photopolymerization of BisGMA-based dimethacrylates generates highly crosslinked, glassy networks that are structurally heterogeneous and highly brittle , while also being prone to hydrolytic and enzymatic degradation in moist environments due to the presence of ester functionalities in the monomer structures . In addition, the low gel point conversion that occurs in a chain-growth polymerization of dimethacrylate resins necessitates that significant polymerization-induced shrinkage stress will arise while the relatively low conversion at which vitrification occurs limits the maximum conversion and may lead to significant amount of residual monomer . The shrinkage and stress development can lead to micro-cracks, adhesive failures, and secondary caries . Over the past decade, alternative chemistries/mechanisms to minimize or eliminate the detrimental characteristics associated with traditional methacrylate-based dental resins have been explored. Ring-opening polymerizations utilizing epoxy, silorane, or cyclopropyl functional groups in resins as well as on nanofillers generated low volumetric shrinkage , and step-growth polymerization of thiol-ene or CuAAC (copper(I)-catalyzed azide-alkyne cycloaddition) resins achieved reduced polymerization shrinkage stress via delayed gelation . In addition, hybrid polymerizations of ternary systems including chain-growth polymerization of methacrylates with step-growth polymerization of thiol-ene/thiol-yne, produced interpenetrating polymer networks (IPNs) with a dramatic reduction in shrinkage stress . Further, incorporation of allyl sulfide or trithiocarbonate functionalities in dimethacrylate-based or other polymerized networks effectively lowered polymerization shrinkage stress via addition-fragmentation chain transfer .
Previously, we introduced the photo-initiated copper(I)-catalyzed azide-alkyne cycloaddition (photo-CuAAC) polymerization ( Scheme 1 ) as a means to prepare a glassy thermoset with significantly reduced polymerization shrinkage stress at complete conversion while simultaneously maintaining improved mechanical performance . The preeminent benefits of the photo-CuAAC polymers over conventional BisGMA-based dimethacrylate polymers lie not only in the nature of the step-growth polymerization and the associated delayed gel point conversion, which contributes to reduced shrinkage stress , but also on the formation of rigid triazole linkages as a product that significantly enhance the overall mechanical behavior . Furthermore, owing to the “click” chemistry of a highly orthogonal CuAAC reaction without forming a byproduct , the kinetics and mechanics of the CuAAC resin-based crosslinked photopolymers have been explored with varying monomer structures, photoinitiators, copper salts, and/or light exposure conditions . Herein, we investigate polymerization kinetics coupled with in situ measurement of reaction temperature and (thermo-) mechanical properties of photo-polymerized CuAAC composites. These experimental formulations with various silica microfiller compositions use visible light photo-activation to achieve rapid photo-curable materials with excellent mechanical performance. For dental restorative applications, polymerization shrinkage stress, flexural modulus (or Young’s modulus), flexural strength, and flexural toughness are considered as essential characteristics in dental composites, and these are compared between CuAAC composites and a BisGMA-based dimethacrylate composite used as a control.

2
Experimental section
2.1
Materials
1,3-Bis(2-isocyanatopropan-2-yl)benzene, dibutyltin dilaurate, tetrahydrofuran, 6-chloro-1-hexanol, sodium azide, 1,1,1-tris(hydroxymethyl)propane, propargyl bromide, propargyl alcohol, 3-(triethoxysilyl)propyl isocyanate, copper(II) chloride, N , N , N ′, N ′, N ″-pentamethyldiethylenetriamine (PMDETA), camphorquinone (CQ), ethyl 4-(dimethylamino)benzoate (EDAB), toluene, and acetonitrile were used as received from Sigma–Aldrich. Propylamine, sodium hydroxide, dimethyl sulfoxide, dimethylformamide, methanol, and sodium sulfate were used as received from Fisher Scientific. The BisGMA/TEGDMA (70/30) comonomer mixture was used as donated from ESSTECH. Fusion silane coupling agent was used as received from George Taub Products & Fusion Co., Inc. Schott glass (mean particle size of 0.4 μm) with both untreated surface and surface treated with γ–methacryloxypropyltrimethoxysilane were used as received from ESSTECH. Bis(6-azidohexyl) (1,3-phenylenebis(propane-2,2-diyl))dicarbamate (BZ-AZ), 1-(prop-2-yn-1-yloxy)-2,2-bis((prop-2-yn-1-yloxy)methyl)butane (AK), and prop-2-yn-1-yl (3-(triethoxysilyl)propyl)carbamate (Si-AK) were synthesized according to previously reported procedures . All synthetic procedures and NMR predictions are provided in the Supporting information. Azides were synthesized according to the azide safety rules and handled with appropriate precaution when working with monomers, resins, and polymers in small quantities ( Fig. 1 ).
2.2
Methods
2.2.1
BisGMA/TEGDMA composite preparation
A BisGMA:TEGDMA (70:30 weight ratio) mixture with 0.6 wt% CQ and 1.6 wt% EDAB was prepared by physical mixing. Methacrylated microfillers (Schott, 0.4 μm) were added to the resin mixture and blended in a speedmixer (DAC 150 FVZ, Flakteck) to ensure uniform dispersion of the fillers.
2.2.2
CuAAC composites preparation
Stoichiometric mixtures of di-azide and tri-alkyne (a mole ratio of 1:1 to azide:alkyne) with 2 mole percentage of CuCl 2 [PMDETA] per functionality, 0.6 wt% CQ, 1.6 wt% EDAB were prepared. Methanol was added to homogenize the mixture and later removed in vacuo . The residual solvent content of each resin was verified by 1 H-NMR using a Bruker Avance-III 400 MHz spectrometer with 16 scans and 1 s of relaxation time. For all experiments, the residual methanol concentration was <0.5 wt%. Alkyne-functionalized microfillers (Schott, 0.4 μm) were added to the resin mixture and blended in a speedmixer (DAC 150 FVZ, Flakteck) to ensure uniform dispersion of fillers.
2.2.3
Filler functionalization
Glass particles (20 g; Schott, 0.4 μm) were placed in a glass tube and dried for 3.5 h at 180 °C in vacuo using a Buchi drying glass oven. To a round bottom flask containing 400 ml of anhydrous toluene, 6 ml of prop-2-yn-1-yl (3-(triethoxysilyl)propyl)carbamate (Si-AK), 0.4 g of propylamine, and 20 g of the dried microparticles were added while purging under nitrogen. The reaction mixture was stirred at 75 °C overnight to accommodate the silanization process. The liquid suspension was centrifuged 4 times with excess toluene (3000 rpm for 5 min) followed by centrifuging 3 times with methylene chloride in dilution (3000 rpm for 15 min). The solid pellets were collected after each centrifugation cycle and redispersed in excess solvent for the additional cycles. The final washed filler particles were dried in vacuo at 70 °C overnight and 100 °C for 2 h. Thermogravimetry (TG) and diffuse reflectance infrared Fourier transform spectroscopy (DRIFTS) were used to analyze the functionalized particles (see Figs. S1, S2).
2.2.4
Fourier transform infrared spectroscopy
An FTIR spectrometer (Nicolet 6700) connected to a tensometer via fiber optic cables was used to monitor the real-time polymerization kinetics in concert with stress measurements. Samples were placed between two cylindrical quartz rods, and 600 mW cm −2 of light was irradiated from the bottom rod using a light guide connected to a mercury lamp (Acticure 4000, EXFO) with 400–500 nm bandgap filter. A radiometer (Model 100, Demetron Research) was used to measure the light intensity transmitted from the end of the quartz rod. The overtone signal of the alkyne was monitored between 6538–6455 cm −1 , and the overtone signal of the methacrylate was measured between 6250–6096 cm −1 .
2.2.5
Polymerization shrinkage stress measurement
A tensometer (American Dental Association Health Foundation, ADAHF-PRC) was utilized to monitor polymerization post-gel shrinkage stress using cantilever beam deflection theory with in situ polymerization kinetic measurements . The sample (6 mm in diameter, 1 mm in thickness) was placed in a cavity between two cylindrical quartz rods, which were previously treated with silane (methacrylate silane coupling agent or Si-AK) to improve the interfacial adhesion, and sealed with a polytetrafluoroethylene (PTFE) sleeve to prevent oxygen inhibition. 600 mW cm −2 of light was transmitted through the bottom rod using a light guide connected to a mercury lamp (Acticure 4000, EXFO) with a 400–500 nm bandgap filter. The deflection of the aluminum beam, caused by a tensile force exerted with bonded sample shrinkage, was measured with a linear variable differential transformer (LVDT) and converted to stress based upon beam calibration constant and cross-sectional area of the sample. A simultaneous measurement of functional group conversion with polymerization shrinkage stress by fiber optic cables was recorded for 15 min.
2.2.6
Dynamic mechanical analysis
A DMA Q800 (TA instruments) in multi-frequency-strain mode with a frequency of 1 Hz and a heating rate of 3 °C min −1 was used to measure the storage modulus and the glass transition temperature (T g ), which was taken as the peak of the tan δ (a ratio of E”/E’: the storage and loss moduli) curve. An irradiance of ∼300 mW cm −2 at 455 nm from a light emitting diode (LED Thorlabs) was directed to one side of the sample for 60 s at room temperature followed immediately by an analogous cure on the opposite side of the sample. The rectangular dimensions of each sample specimen were 0.25 × 5 × 25 mm (t × w × l). The samples were placed in the oven at 70 °C overnight and 150 °C for 1 h.
2.2.7
Three-point flexural test
Three-point bending (MTS 858 Mini Bionix II) with a strain rate of 1 mm min −1 and a span of 20 mm was used to obtain flexural modulus, flexural strength, and flexural toughness. Flexural modulus was calculated from the initial slope between 0.5 and 1% strain, and flexural strength was obtained using the following equation: <SPAN role=presentation tabIndex=0 id=MathJax-Element-1-Frame class=MathJax style="POSITION: relative" data-mathml='σ=3FL2BH2′>σ=3FL2BH2σ=3FL2BH2
σ = 3 FL 2 BH 2
, where F is the maximum load, L is the length of span, B is the width of the sample specimen, H is the height of the sample specimen . Photo-activation was conducted at ∼300 mW cm −2 of 455 nm light from an LED (Thorlabs). The irradiation was conducted for 60 s at room temperature on one side of the sample followed by immediate inversion to cure for 60 s on the opposite side of the sample. The samples were placed in the oven at 70 °C overnight and 150 °C for 30 min and used for the experiment within 1 day after the thermal cure. The rectangular dimensions of each sample specimen were 2 × 2 × 25 mm (t × w × l).
2.2.8
Viscosity measurement
A rheometer (ARES, TA Instruments) was used to measure the viscosity of the monomers in the torque ranges from 2 × 10 −6 to 2 × 10 −2 N m, using 200 μm thick samples placed between 8 mm diameter quartz plates.
2.2.9
Thermal gravimetric analysis
Thermogravimetric analysis (TGA Pyris 1, PerkinElmer) was used to analyze the functionalized fillers. Each sample was run in a nitrogen atmosphere (20 ml min −1 ) from 50 °C to 850 °C at a heating rate of 10 °C min −1 .
2.2.10
Statistical analysis
Statistical analysis of the experiments was performed via one-way analysis of variance (ANOVA), and multiple pair-wise comparisons were conducted via a Tukey’s post-hoc test with a significance level of 0.05. The number of repetitions for each experiment were as follows: dynamic mechanical analysis (n = 3), polymerization shrinkage stress (n = 3), three-point flexural test (n = 5), and viscosity measurement (n = 3).
2
Experimental section
2.1
Materials
1,3-Bis(2-isocyanatopropan-2-yl)benzene, dibutyltin dilaurate, tetrahydrofuran, 6-chloro-1-hexanol, sodium azide, 1,1,1-tris(hydroxymethyl)propane, propargyl bromide, propargyl alcohol, 3-(triethoxysilyl)propyl isocyanate, copper(II) chloride, N , N , N ′, N ′, N ″-pentamethyldiethylenetriamine (PMDETA), camphorquinone (CQ), ethyl 4-(dimethylamino)benzoate (EDAB), toluene, and acetonitrile were used as received from Sigma–Aldrich. Propylamine, sodium hydroxide, dimethyl sulfoxide, dimethylformamide, methanol, and sodium sulfate were used as received from Fisher Scientific. The BisGMA/TEGDMA (70/30) comonomer mixture was used as donated from ESSTECH. Fusion silane coupling agent was used as received from George Taub Products & Fusion Co., Inc. Schott glass (mean particle size of 0.4 μm) with both untreated surface and surface treated with γ–methacryloxypropyltrimethoxysilane were used as received from ESSTECH. Bis(6-azidohexyl) (1,3-phenylenebis(propane-2,2-diyl))dicarbamate (BZ-AZ), 1-(prop-2-yn-1-yloxy)-2,2-bis((prop-2-yn-1-yloxy)methyl)butane (AK), and prop-2-yn-1-yl (3-(triethoxysilyl)propyl)carbamate (Si-AK) were synthesized according to previously reported procedures . All synthetic procedures and NMR predictions are provided in the Supporting information. Azides were synthesized according to the azide safety rules and handled with appropriate precaution when working with monomers, resins, and polymers in small quantities ( Fig. 1 ).
2.2
Methods
2.2.1
BisGMA/TEGDMA composite preparation
A BisGMA:TEGDMA (70:30 weight ratio) mixture with 0.6 wt% CQ and 1.6 wt% EDAB was prepared by physical mixing. Methacrylated microfillers (Schott, 0.4 μm) were added to the resin mixture and blended in a speedmixer (DAC 150 FVZ, Flakteck) to ensure uniform dispersion of the fillers.
2.2.2
CuAAC composites preparation
Stoichiometric mixtures of di-azide and tri-alkyne (a mole ratio of 1:1 to azide:alkyne) with 2 mole percentage of CuCl 2 [PMDETA] per functionality, 0.6 wt% CQ, 1.6 wt% EDAB were prepared. Methanol was added to homogenize the mixture and later removed in vacuo . The residual solvent content of each resin was verified by 1 H-NMR using a Bruker Avance-III 400 MHz spectrometer with 16 scans and 1 s of relaxation time. For all experiments, the residual methanol concentration was <0.5 wt%. Alkyne-functionalized microfillers (Schott, 0.4 μm) were added to the resin mixture and blended in a speedmixer (DAC 150 FVZ, Flakteck) to ensure uniform dispersion of fillers.
2.2.3
Filler functionalization
Glass particles (20 g; Schott, 0.4 μm) were placed in a glass tube and dried for 3.5 h at 180 °C in vacuo using a Buchi drying glass oven. To a round bottom flask containing 400 ml of anhydrous toluene, 6 ml of prop-2-yn-1-yl (3-(triethoxysilyl)propyl)carbamate (Si-AK), 0.4 g of propylamine, and 20 g of the dried microparticles were added while purging under nitrogen. The reaction mixture was stirred at 75 °C overnight to accommodate the silanization process. The liquid suspension was centrifuged 4 times with excess toluene (3000 rpm for 5 min) followed by centrifuging 3 times with methylene chloride in dilution (3000 rpm for 15 min). The solid pellets were collected after each centrifugation cycle and redispersed in excess solvent for the additional cycles. The final washed filler particles were dried in vacuo at 70 °C overnight and 100 °C for 2 h. Thermogravimetry (TG) and diffuse reflectance infrared Fourier transform spectroscopy (DRIFTS) were used to analyze the functionalized particles (see Figs. S1, S2).
2.2.4
Fourier transform infrared spectroscopy
An FTIR spectrometer (Nicolet 6700) connected to a tensometer via fiber optic cables was used to monitor the real-time polymerization kinetics in concert with stress measurements. Samples were placed between two cylindrical quartz rods, and 600 mW cm −2 of light was irradiated from the bottom rod using a light guide connected to a mercury lamp (Acticure 4000, EXFO) with 400–500 nm bandgap filter. A radiometer (Model 100, Demetron Research) was used to measure the light intensity transmitted from the end of the quartz rod. The overtone signal of the alkyne was monitored between 6538–6455 cm −1 , and the overtone signal of the methacrylate was measured between 6250–6096 cm −1 .
2.2.5
Polymerization shrinkage stress measurement
A tensometer (American Dental Association Health Foundation, ADAHF-PRC) was utilized to monitor polymerization post-gel shrinkage stress using cantilever beam deflection theory with in situ polymerization kinetic measurements . The sample (6 mm in diameter, 1 mm in thickness) was placed in a cavity between two cylindrical quartz rods, which were previously treated with silane (methacrylate silane coupling agent or Si-AK) to improve the interfacial adhesion, and sealed with a polytetrafluoroethylene (PTFE) sleeve to prevent oxygen inhibition. 600 mW cm −2 of light was transmitted through the bottom rod using a light guide connected to a mercury lamp (Acticure 4000, EXFO) with a 400–500 nm bandgap filter. The deflection of the aluminum beam, caused by a tensile force exerted with bonded sample shrinkage, was measured with a linear variable differential transformer (LVDT) and converted to stress based upon beam calibration constant and cross-sectional area of the sample. A simultaneous measurement of functional group conversion with polymerization shrinkage stress by fiber optic cables was recorded for 15 min.
2.2.6
Dynamic mechanical analysis
A DMA Q800 (TA instruments) in multi-frequency-strain mode with a frequency of 1 Hz and a heating rate of 3 °C min −1 was used to measure the storage modulus and the glass transition temperature (T g ), which was taken as the peak of the tan δ (a ratio of E”/E’: the storage and loss moduli) curve. An irradiance of ∼300 mW cm −2 at 455 nm from a light emitting diode (LED Thorlabs) was directed to one side of the sample for 60 s at room temperature followed immediately by an analogous cure on the opposite side of the sample. The rectangular dimensions of each sample specimen were 0.25 × 5 × 25 mm (t × w × l). The samples were placed in the oven at 70 °C overnight and 150 °C for 1 h.
2.2.7
Three-point flexural test
Three-point bending (MTS 858 Mini Bionix II) with a strain rate of 1 mm min −1 and a span of 20 mm was used to obtain flexural modulus, flexural strength, and flexural toughness. Flexural modulus was calculated from the initial slope between 0.5 and 1% strain, and flexural strength was obtained using the following equation: <SPAN role=presentation tabIndex=0 id=MathJax-Element-2-Frame class=MathJax style="POSITION: relative" data-mathml='σ=3FL2BH2′>σ=3FL2BH2σ=3FL2BH2
σ = 3 FL 2 BH 2
, where F is the maximum load, L is the length of span, B is the width of the sample specimen, H is the height of the sample specimen . Photo-activation was conducted at ∼300 mW cm −2 of 455 nm light from an LED (Thorlabs). The irradiation was conducted for 60 s at room temperature on one side of the sample followed by immediate inversion to cure for 60 s on the opposite side of the sample. The samples were placed in the oven at 70 °C overnight and 150 °C for 30 min and used for the experiment within 1 day after the thermal cure. The rectangular dimensions of each sample specimen were 2 × 2 × 25 mm (t × w × l).
2.2.8
Viscosity measurement
A rheometer (ARES, TA Instruments) was used to measure the viscosity of the monomers in the torque ranges from 2 × 10 −6 to 2 × 10 −2 N m, using 200 μm thick samples placed between 8 mm diameter quartz plates.
2.2.9
Thermal gravimetric analysis
Thermogravimetric analysis (TGA Pyris 1, PerkinElmer) was used to analyze the functionalized fillers. Each sample was run in a nitrogen atmosphere (20 ml min −1 ) from 50 °C to 850 °C at a heating rate of 10 °C min −1 .
2.2.10
Statistical analysis
Statistical analysis of the experiments was performed via one-way analysis of variance (ANOVA), and multiple pair-wise comparisons were conducted via a Tukey’s post-hoc test with a significance level of 0.05. The number of repetitions for each experiment were as follows: dynamic mechanical analysis (n = 3), polymerization shrinkage stress (n = 3), three-point flexural test (n = 5), and viscosity measurement (n = 3).
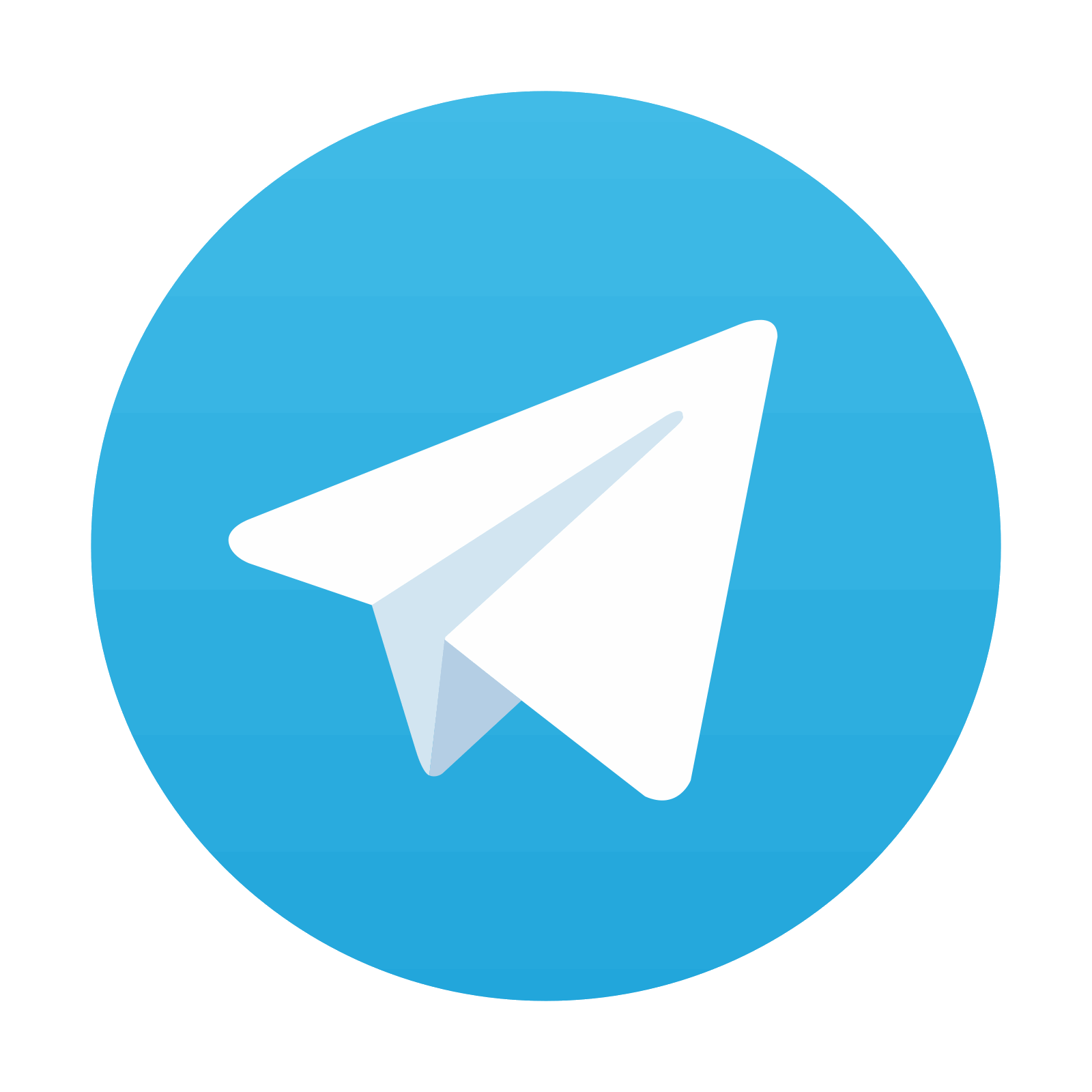
Stay updated, free dental videos. Join our Telegram channel

VIDEdental - Online dental courses
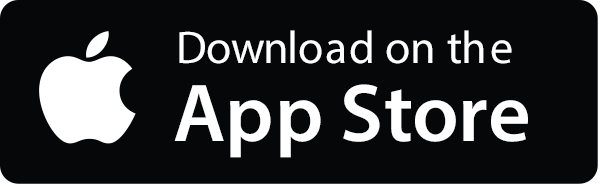
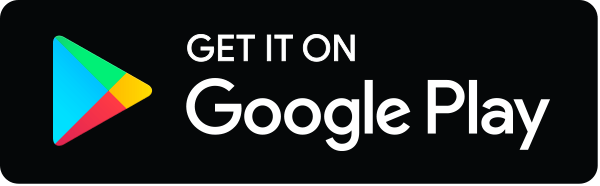